Professor Ard Louis is a theoretical physicist here at the Rudolf Peierls Centre for Theoretical Physics. The department recently hosted the oxDNA users and developers workshop 2024. Here he tells the story of oxDNA, the most successful and widely adopted coarse-grained model for DNA self-assembly.
The magic of self-assembly
The cells in your body are packed with exquisite nanoscale machines that perform a dizzying array of functions. For instance, each time you flex your biceps, a vast army of myosin motors march in lockstep along actin fibre tracks, contracting your muscle cells. Each motor generates about 1pN of force, so you need on the order of a trillion myosin motors working in unison just to lift a football. Meanwhile, another molecular motor, ATP synthase, harnesses a proton gradient to power its rotating turbines, converting electrical energy into the ATP molecules that fuel your myosin armies.
If such biological machines were scaled up a hundred million fold to make them visible to the naked eye, we’d assume they were built in factory assembly lines. But that’s not what happens. Instead, buffeted by thermal motion and without external guidance, individual proteins spontaneously self-assemble into intricate three-dimensional structures. It’s akin to tossing Lego blocks and glue into a box, giving it a shake, and somehow ending up with a perfectly assembled train.
How does nature pull off such mind-boggling feats? How can it overcome the entropic challenge of precisely assembling a desired structure amidst countless incorrect possibilities? The solution to a closely related needle-in-a-haystack problem – predicting the shapes into which proteins fold (self-assemble) from a linear chain of connected units—was the focus of this year’s Nobel Prize in Chemistry. The phenomenon of self-assembly opens up a world of intriguing questions in nonequilibrium statistical mechanics. There are deep connections to the mathematics of search and optimisation. Beyond its theoretical allure, self-assembly is of immense practical significance, as it is fundamental to creating anything at the nanoscale.
Self-assembly with DNA
For many years my colleague Professor Jonathan Doye from Oxford Theoretical Chemistry, and I have collaborated on theories of self-assembly. When I joined the Physics department in 2006, Professor Andrew Turberfield and Dr Jonathan Bath, along with other key researchers, were pioneering the self-assembly of DNA nanostructures.
By leveraging the precise base-pairing rules of DNA—where adenine (A) pairs with thymine (T), and cytosine (C) pairs with guanine (G)—they demonstrated that short synthetic strands of DNA could be programmed to 'self-assemble' into nearly any geometric shape in both two and three dimensions. Moreover, Andrew’s group also engineered artificial analogs of nature’s autonomous nanomachines such as DNA based linear walkers on tracks (see Figure 1), showcasing the incredible potential of dynamic DNA nanotechnology.
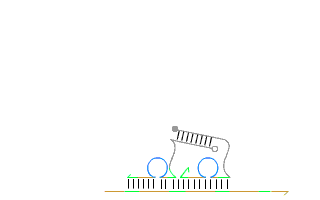
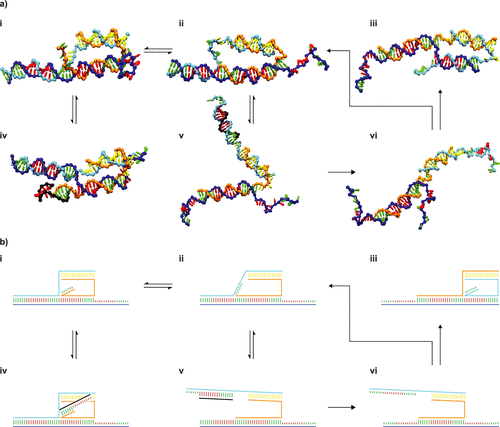
These breakthroughs marked a step-change in what artificial self-assembly could achieve. Early results on self-assembly with RNA, a related, but more temperamental molecule, are showing significant potential. In recent years, this field has expanded at an astonishing pace fuelled by the promise of applications in drug delivery, molecular computing, and biosensing. Today, numerous spin-off companies are racing to commercialise this groundbreaking research.
The birth of oxDNA
The ability to precisely control DNA self-assembly also makes it an ideal playground for exploring the fundamental physics of self-assembly. While Jonathan and I primarily worked with abstract models, the experimental breakthroughs at Oxford inspired us to develop a computational model of DNA self-assembly that could be efficiently simulated. Initially, our goal was to explore the fundamental principles of assembly.
Together with DPhil student Iain Johnston (now a group leader at the University of Bergen) and MPhys student Tom Ouldridge (now a group leader at Imperial College), we began by creating a simple linear polymer model with sequence-dependent binding interactions. This model offered a qualitative explanation of the core physics driving DNA assembly.
Tom decided to stay for a DPhil in order to push these ideas further and produce a more sophisticated model of DNA. His thesis culminated in the creation of oxDNA, a coarse-grained model that captures key physical processes such as base-pairing, stacking interactions, and strand flexibility. These features are crucial for understanding DNA hybridisation, folding, strand displacement, and the dynamics of fully assembled DNA structures.
A critical aspect of his approach was the incorporation of as much emergent physics as possible at each step. For instance, DNA’s iconic double helix does not arise from imposed angular constraints but from an intrinsic mismatch in length scales. The bases prefer to stack at a distance of 3.3 Å, but along the backbone they are spaced 6 Å apart. To resolve this discrepancy, DNA twists and bends its backbone, incurring an elastic energy cost to recover stacking energy between the bases. Accurately capturing such physical mechanisms is essential to model the transition between single- and double-stranded DNA, which lie at the heart of DNA nanotechnology.
Our team soon expanded with many talented DPhil students including Petr Šulc, (now group leader at the University of Arizona and the Technical University Munich), Christian Matek (now a group leader at the University of Erlangen), Ferdinando Randisi (co-founder of Fabric Nano), Ryan Harrison (now a Presidential Innovation Fellow, Centers for Disease Control and Prevention), Ben Snodin (now at the UK AI Safety Institute), Megan Engel (now a group leader at the University of Calgary), Wilber Lim (now Director of the National Quantum Office in Singapore), Hemani Chhabdra (now a postdoc at UIUC), Hannah Fowler (now an editor at the RSC) Michael Selby (now at Signaloid), Domen Presern (now in the wine industry) and theory DPhils co-supervised and embedded in Andrew’s experimental group including Joakim Bohlin (now at Chalmers University), Behnam Najafi (now at Aurora energy), and Eryk Ratajczyk (still finishing his DPhil) and postdocs Flavio Romano (now group leader at the University of Venice), Lorenzo Rovigatti (now group leader at the University of Rome) Majid Mosayebi (now at Oxford Nanopore) and John Schreck (now at the National Center for Atmospheric Research·in Boulder CO). Together, this crack team wrote new versions of oxDNA, developed a similar model for RNA (oxRNA) and released the code publicly – a lot of work.
Unexpected applications
Our original goal was to explore the basic physics of DNA self-assembly. At the start of the project, we would have been thrilled if oxDNA qualitatively captured the behaviour of self-assembled DNA structures, allowing us to study fundamental self-assembly processes. That it did, and we were able to work out, and are still working on, many fascinating and subtle mechanisms of biophysical assembly. However, oxDNA also far exceeded our expectations for accuracy, often demonstrating near-quantitative agreement with experimental results.
The unexpected accuracy has made oxDNA the go-to tool for prototyping and analysing new experimental designs, used by hundreds of academic and industrial laboratories worldwide. It is now the most successful and widely adopted coarse-grained model for DNA self-assembly. Interestingly, we never intended for oxDNA to achieve such practical relevance – we didn’t think that goal was remotely feasible. oxDNA exemplifies the power of curiosity-driven, blue-sky research to yield unanticipated and transformative applications.
oxDNA: the future
There is a vibrant international community of scientists working on extending and improving oxDNA. In September, 50 researchers gathered in Oxford for an oxDNA users and developers workshop to chart the next chapter. While Jonathan and I remain involved, leadership has transitioned to an exciting new generation of scientists.
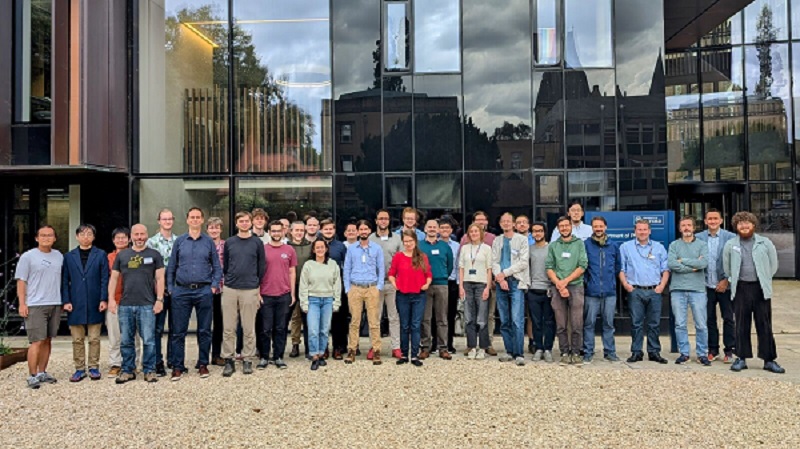
For instance, Oliver Henrich’s group at the University of Strathclyde is spearheading the development of oxDNA3, which will incorporate sequence-dependent elasticity. This innovation tackles a grand challenge in biology: deciphering an 'elastic code' that complements DNA’s digital genetic code by influencing how genetic information is read and regulated. Meanwhile, Megan Engel, in collaboration with Michael Brenner’s team at Harvard, is leveraging machine learning to automate the development of new force fields for oxDNA, which should accelerate our ability to improve and fine-tune future versions of oxDNA and oxRNA.
The heart of the oxDNA infrastructure development is now mainly in Arizona, Munich and Rome, where Petr Šulc and Lorenzo Rovigatti lead the overall coding efforts. At the Oxford workshop, Petr announced a new $1.4M NSF POSE grant to expand the oxDNA open source ecosystem. The future of oxDNA is bright.
[1] Coordinated chemomechanical cycles: a mechanism for autonomous molecular motion, SJ Green et al, Physical review letters 101 238101 (2008)
[2] Optimizing DNA nanotechnology through coarse-grained modeling: a two-footed DNA walker, Thomas E Ouldridge et al, ACS Nano 7, 2479 (2013)