Introduction
When an intense laser pulse propagates through a plasma, the ponderomotive force pushes electrons away from the front and back of the pulse thereby forming a trailing longitudinal density wave. The longitudinal electric field in the plasma wave can be as high as 100 kilovolts per micron, more than three orders of magnitude larger than that found in conventional RF accelerators such as those used at CERN. Particles injected into the correct phase of the plasma wave can be accelerated to energies of order 1 GeV in only a few centimetres. This 'laser wakefield accelerator' is particularly promising for generating beams of short pulse, high-energy electrons for applications in femtosecond electron diffraction, medical imaging, and miniature free-electron X-ray lasers.
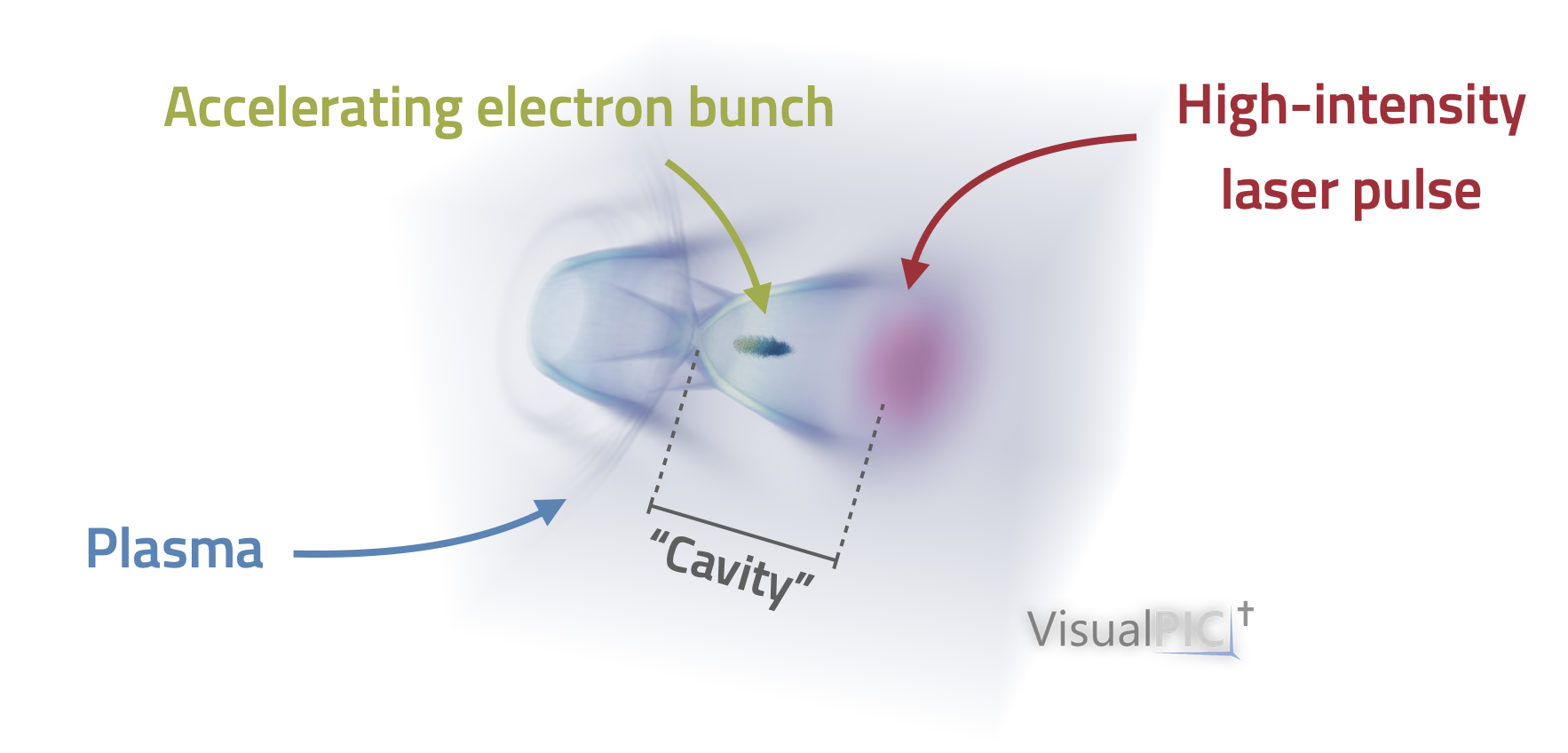
One factor which limits the energy to which particles can be accelerated is the distance over which the intensity of the driving laser can be maintained. Our group has developed several techniques for channelling laser pulses with peak intensities of up to 1018 Wcm-2 over distances which are much longer than the limit set by diffraction. In collaboration with a group at Lawrence Berkeley National Laboratory (LBNL), we have used these high-intensity waveguides to extend the length over which acceleration can be maintained by an order of magnitude and thereby generated electrons with energies of 1 GeV. This energy is of the order of that used in many synchrotrons around the world, but the plasma accelerator is only 33 mm long instead of 150 m!
The longer term goal of our work is the development of controlled, multi-GeV plasma accelerators capable of high (i.e. kHz) repetition rate operation. We are therefore investigating the use of novel laser technologies to drive the plasma wave; all-optical "indestructible" plasma waveguides; and methods for controlling the injection of electrons into the plasma wakefield.
Further reading
- S. M. Hooker, "Developments in laser-driven plasma accelerators," Nature Photonics 7 775–782 (2013). DOI: 10.1038/nphoton.2013.234
- W. P. Leemans, S. M. Hooker et al.[/url], "GeV electron beams from a centimetre-scale accelerator," Nature Physics 2 696 (2006). DOI: 10.1038/nphys418
- J. Osterhoff, S. Karsch, S. M. Hooker et al.[/url], "Generation of Stable, Low-Divergence Electron Beams by Laser-Wakefield Acceleration in a Steady-State-Flow Gas Cell," Phys. Rev. Lett. 101 085002 (2008). DOI: 10.1103/PhysRevLett.101.085002
- T.P. Rowlands-Rees, S. M. Hooker et al., "Laser-Driven Acceleration of Electrons in a Partially Ionized Plasma Channel," Phys. Rev. Lett. 100 105005 (2008). DOI: 10.1103/PhysRevLett.100.105005]
Acceleration in waveguides
In order to reach a laser intensity required to drive a laser-plasma accelerator, which is of order 1018 Wcm-2, it is necessary to focus the laser to a spot size of a few tens of micrometers. However, a focused laser pulse will diffract in a distance scale of order the Rayleigh range \(z_R = \pi w_0^2 / \lambda\), where \(\lambda\) is the laser wavelength. This distance is only a millimetre or so for laser wavelengths \(\lambda \approx 1 \mathrm{\mu m}\), and hence some means for keeping the laser focused over the length of the accelerator is necessary.
A general method to guide optical radiation is to use a system in which the refractive index decreases with radial distance from the propagation axis. This is known as a gradient refractive index (GRIN) waveguide, and it is the basis of one type of optical fibre. Of course, standard optical fibres cannot be used at the intensities relevant to laser-plasma accelerators, since it would be destroyed in a single shot! However, the same approach can be used if the "fibre" is made from plasma, since (provided the plasma is fully ionized) this cannot be destroyed by the laser pulse.
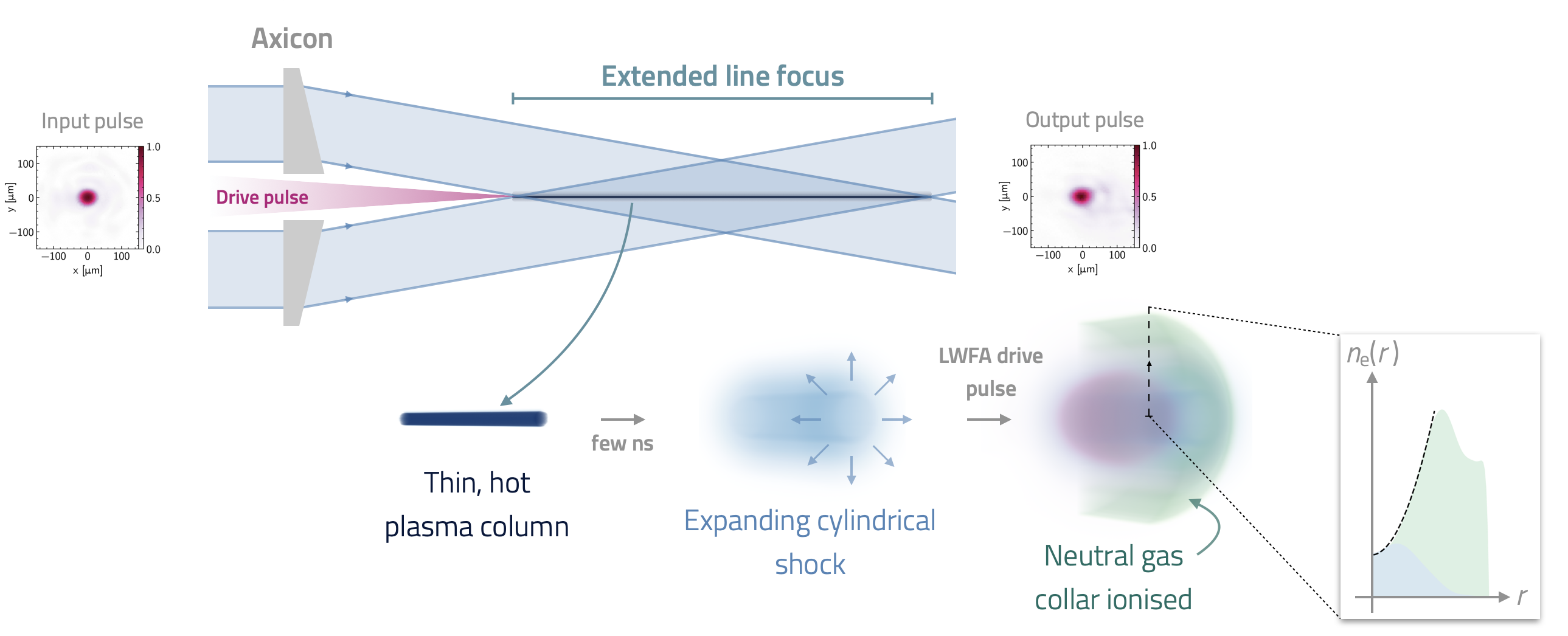
A large variety of methods have been developed for creating "plasma channels" of this type. One of these, the gas-filled capillary discharge waveguide, was developed by our group, and is now used widely in plasma accelerator research. Indeed it was used in the first experiments to generate GeV electron beams in a laser-driven accelerator.
More recently we have been studying all-optical methods for generating plasma channels, as first developed by Prof. Howard Milchberg at the University of Maryland. In this approach a column of hot plasma is generated by an axicon lens (a conical lens). If it is formed quickly, and it is sufficiently hot, the plasma column will rapidly expand radially, driving a shock wave into the surrounding cold gas. The density of the plasma between the axis of the original column and the shock front increases with distance from the axis, i.e. it is a plasma channel.
A major advantage of this approach is that the plasma channels are free-standing, and as such are immune to damage by a mis-directed laser pulse. The development of "indestructible" plasma channels of this type is important for real-world applications of laser-plasma accelerators, especially those operating at high pulse repetition rates.
The first all-optical plasma channels of this type used picosecond-duration laser pulses to generate and heat the initial plasma column. However, since the heating occurred through laser-driven collisions, this method was limited to relatively high plasma densities. We have developed an alternative approach, which allows the creation of plasma channels at lower densities (and hence acceleration to higher particle energies). In this approach the initial plasma column is formed and heated by optical field ionization (OFI), in which the very high field of the channel-forming laser ionizes the target gas atoms by distorting the atomic potential binding the valance electrons. The ionized electrons are driven by the laser field, and can gain energy from it. Importantly, this electron heating is independent of the plasma density, and hence it is possible to generate hot, low density plasma columns, which expand to generate plasma channels with low on-axis densities. In recent work we have demonstrated guiding of high-intensity laser pulses in hydrodynamic optical-field-ionized (HOFI) plasma channels 100 mm long.
Further reading
- A. Alejo, J. Cowley, A. Picksley, R. Walczak, and S. M. Hooker, "Demonstration of Kilohertz Operation of Hydrodynamic Optical-Field-Ionized Plasma Channels," Phys. Rev. Accel. Beams 25 011301 (2022). DOI: https://doi.org/10.1103/physrevaccelbeams.25.011301
- A Picksley, A Alejo, R Shalloo, C Arran, A von Boetticher, L Corner, J Holloway, J Jonnerby, O Jakobsson, C Thornton, R Walczak, and S. M. Hooker, "Meter-scale conditioned hydrodynamic optical-field-ionized plasma channels," Phys. Rev. E 10253201 (2020). DOI: https://doi.org/10.1103/PhysRevE.102.053201
- RJ Shalloo, C Arran, A Picksley, A von Boetticher, L Corner, J Holloway, G Hine, J Jonnerby, HM Milchberg, C Thornton, R Walczak, and SM Hooker, "Low-density hydrodynamic optical-field-ionized plasma channels generated with an axicon lens," Phys. Rev. Accel. Beams 22 041302 (2019). DOI: https://doi.org/10.1103/PhysRevAccelBeams.22.041302
- RJ Shalloo, C Arran, L Corner, J Holloway, J Jonnerby, R Walczak, HM Milchberg, and S. M. Hooker, "Hydrodynamic optical-field-ionized plasma channels, Phys. Rev. E 97 053203 (2018). DOI: https://doi.org/10.1103/PhysRevE.97.053203
Multi-pulse laser wakefield accelerators
The laser wakefield accelerator (LWFA) has many potential applications. However, most of these - including, in the long term, laser-driven particle colliders - will require the accelerator to operate at much higher pulse repetition rates than is possible with the Ti:sapphire lasers used today.
An interesting new approach being developed by a collaboration between groups in the sub-departments of Particle Physics and Atomic & Laser Physics: multi-pulse LWFA, in which a train of low-energy laser pulses drives the plasma wave. If the pulses in the train are spaced by the plasma period then the wakes excited by each pulse interfere coherently to form a large-amplitude wave at the back of the pulse train. The advantage of this approach is that it opens the possibility of using novel laser systems - such as thin-disk and fibre lasers - which can operate at very high pulse repetition rates and with excellent overall efficiency.
We have recently developed a new concept, that we call the Plasma-Modulated Plasma Accelerator (P-MoPA). This exploits the high pulse energy available from a commercial thin-disk laser by converting each picosecond-duration pulse to a train of short pulses that can resonantly excite a plasma wave. As illustrated below, a P-MoPA comprises three stages:
- The `modulator'. A long, high-energy (~ 1 J) `drive' pulse from a thin disk laser is modulated by co-propagating it with the low amplitude plasma wave driven by short, low-energy (~ 100 mJ) `seed' pulse. The drive and seed pulses co-propagate in a long HOFI channel, which causes the drive pulse to develop spectral sidebands spaced by the plasma frequency.
- The `compressor'. Removing the spectral phase acquired by each side-band converts the spectrally-modulated drive pulse to a train of short pulses spaced by the plasma period of the modulator.
- The `accelerator'. Focusing the pulse train into a HOFI channel with the same axial density as that of the modulator resonantly excites a large amplitude wakefield, allowing acceleration to high energies.
We are currently working to demonstrate each of stage of the P-MoPA before seeking to accelerate electrons in a P-MoPA for the first time.
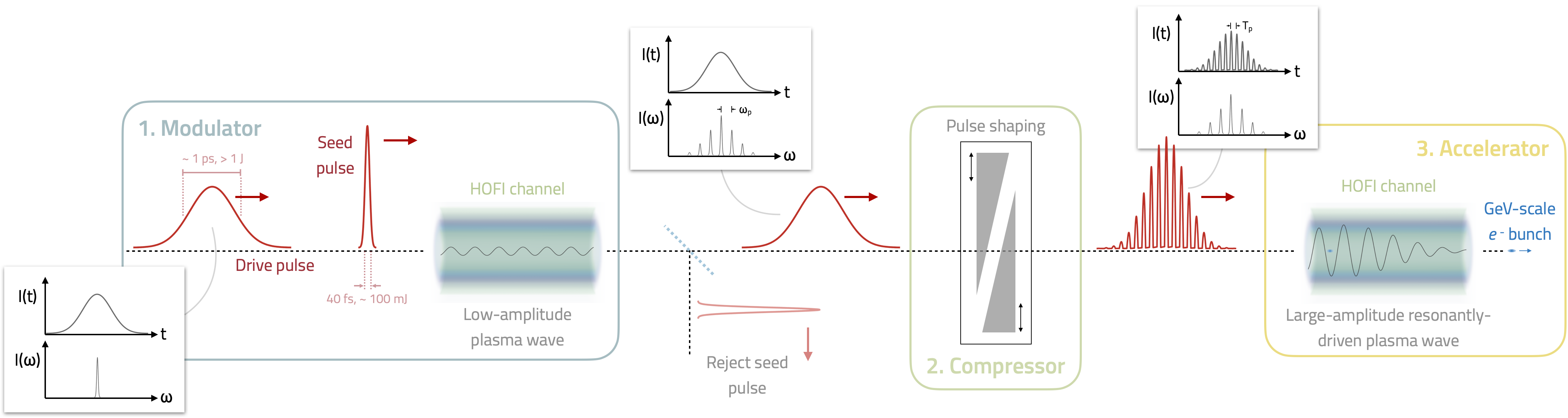
Further reading
- O. Jakobsson, S. M. Hooker, and R. Walczak, "Gev-Scale Accelerators Driven by Plasma-Modulated Pulses from Kilohertz Lasers," Phys. Rev. Lett. 127 184801 (2021). DOI: https://doi.org/10.1103/physrevlett.127.184801
- J. Cowley, C. Thornton, C. Arran, R. J. Shalloo, L. Corner, G. Cheung, C. D. Gregory, S. P. D. Mangles, N. H. Matlis, D. R. Symes, R. Walczak, and S. M. Hooker, "Excitation and Control of Plasma Wakefields by Multiple Laser Pulses," Phys. Rev. Lett. 119 044802 (2017). DOI: 10.1103/PhysRevLett.119.044802
- S. M. Hooker, R. Bartolini, S. P. D. Mangles, A. Tünnermann, L. Corner, J. Limpert, A. Seryi, & R. Walczak, "Multi-Pulse Laser Wakefield Acceleration: A New Route to Efficient, High-Repetition-Rate Plasma Accelerators and High Flux Radiation Sources," J. Phys. B 47 234003 (2014) DOI: 10.1088/0953-4075/47/23/234003
Controlled electron injection
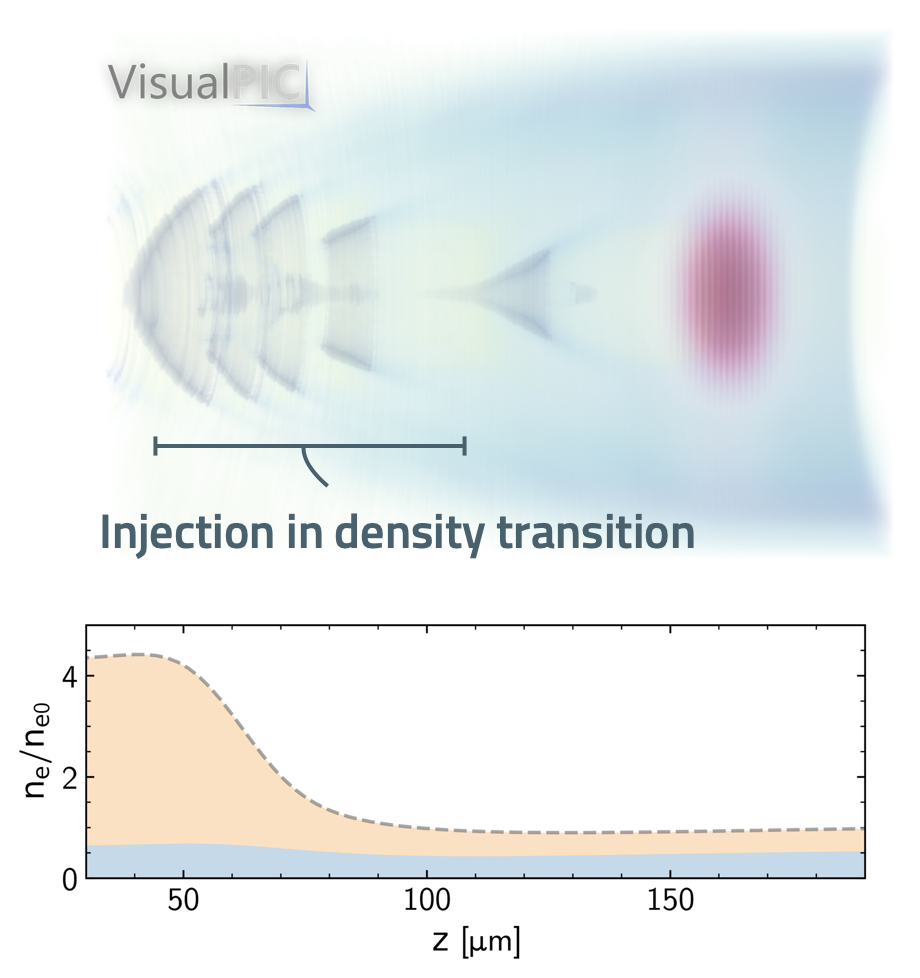
LWFAs have the capability to produce electron bunches from within the background plasma itself, thus eliminating the need for an external electron source. Initial experiments often relied on "self-injection" of electrons, where the plasma is so strongly perturbed by the intense laser pulse that the high-density electron structure at the rear of the accelerating cavity "breaks", with some electrons entering the cavity and being accelerated. However, this process relies on the highly non-linear laser-plasma interaction and hence is very susceptible to fluctuations of the laser pulse or plasma, and hence results in the production of electron bunches with highly-variable properties. We are investigating novel techniques, such as injection via density transitions, to control the process of electron injection into the wakefield and improve the stability of the accelerated electron bunch parameters.
Radiation generation
Radiation can be generated from laser-accelerated beams in two ways. The beams can be passed through an undulator - a periodic array of dipole magnets with alternating orientation - which forces the electrons to oscillate transversely, and hence to radiate. This technique is already used at synchrotron facilities to generate incoherent radiation from THz to x-ray frequencies. If the electron beams are of sufficiently high quality (i.e. have low energy spread and low emittance etc.) then feedback between the generated radiation and the electron bunch causes "micro-bunching" at the radiation wavelength. Radiation emitted in the forward direction by each micro-bunch is in phase, and hence as the degree of micro-bunching increases so does the intensity of the radiation field; as a consequence the intensity of the radiation grows exponentially with propagation through the undulator. X-ray "free-electron lasers" (XFELs), based on this principle, have recently been demonstrated for the first time using kilometre-long conventional accelerators. A long-term goal of our work on laser-driven plasma accelerators is to replace the conventional accelerator with a plasma accelerator only a few centimetres long.
A second method for generating x-rays from laser-accelerated electron beams is to use the transverse oscillation caused by the transverse electric fields in the plasma wave. This oscillation leads to the emission of (broad-band) betatron radiation which can extend to photon energies of tens of keV.
Further reading
- M. Fuchs, F. Gruner, S. Karsch, S. M. Hooker et al., "Laser-driven soft-X-ray undulator source," Nature Physics 5 826 (2009). DOI: 10.1038/NPHYS1404
Laboratory facilities
We have recently moved into new, radiation-shielded high-power laser laboratories. These host an upgraded Ti:sapphire laser system providing 600 mJ, 40 fs pulses at a pulse repetition rate of 10 Hz.
This new facility will allow us to laser-accelerate electrons in Oxford for the first time, and to conduct experiments on novel plasma channels, controlled injection, and multi-pulse wakefield excitation.