Free-energy flows and universal equilibria in turbulent astrophysical plasmas
Supervisors: Alexander Schekochihin, Michael Barnes
In magnetised astrophysical plasmas, there is a turbulent cascade of electromagnetic fluctuations carrying free energy from large to small scales. The energy is typically extracted from large-scale sources (e.g., in the solar wind, the violent activity in the Sun's corona; in accretion discs, the Keplerian shear flow; in galaxy clusters, outbursts from active galactic nuclei) and deposited into heat---the internal energy of ions and electrons. In order for this dissipation of energy to happen, the energy must reach small scales---in weakly collisional plasmas, these are small scales in the 6D kinetic phase space, i.e., what emerges is large spatial gradients of electric and magnetic fields and large gradients of the particle distribution functions with respect to velocities. This prompts two fundamental questions: (1) how does the energy flow through the 6D phase space and what therefore is the structure of the fluctuations in this space: their spectra, phase-space correlation functions etc. [1,2,3] (these fluctuations are best observed in the solar wind, but we can measure density and magnetic fluctuations even in extragalactic plasmas, via X-ray and radio observations); (2) when turbulent fluctuations are dissipated into particle heat, how is their energy partitioned between various species of particles that populate the plasma: electrons, bulk ions, minority ions, fast non-thermal particles (e.g., cosmic rays) [4,5]. The latter question is particularly important for extragalactic plasmas because all we can observe is radiation from the particles and knowing where the internal energy of each species came from is key to constructing and verifying theories both of turbulence and of macroscale dynamics and thermodynamics. This project has an analytical and a numerical dimension (which of these will dominate depends on the student's inclinations). Analytically, we will work out a theory of phase-space cascade at spatial scales between the ion and electron Larmor scales. Numerically, we will simulate this cascade using "gyrokinetic" equations---an approach in which we average over the Larmor motion and calculate the distribution function of "Larmor rings of charge" rather than particles (this reduces the dimension of phase space to 5D, making theory more tractable and numerics more affordable).
With a theory of plasma turbulence in hand, it is possible to attack what is probably the most fundamental question of the field: in the absence of collisions, are there universal equilibria, or classes of equilibria, independent of initial conditions, that a turbulent plasma will want to converge to? There is some recent progress indicating that the answer is yes and that one can predict statistical-mechanically the emergence of universal power-law (in particle energy) distributions [6]---this is exciting both on its own merits and because of the astrophysical challenge of explaining theoretically power-law distributions that are observed for, e.g., cosmic rays or solar-wind electrons. How to construct a theory of that for a magnetised, turbulent plasma is an open and exciting question. Attempting to do this will again involve kinetic theory and/or kinetic simulations.
Background Reading:
- A. A. Schekochihin et al., "Astrophysical gyrokinetics: kinetic and fluid turbulent cascades in magnetized weakly collisional plasmas," Astrophys. J. Suppl. 182, 310 (2009)
- A. A. Schekochihin et al., "Phase mixing vs. nonlinear advection in drift-kinetic plasma turbulence," J. Plasma Phys. 82, 905820212 (2016)
- R. Meyrand et al., "Fluidization of collisionless plasma turbulence," PNAS 116, 1185 (2019)
- Y. Kawazura et al., "Thermal disequilibration of ions and electrons by collisionless plasma turbulence," PNAS 116, 771 (2019)
- J. Squire et al., "High-frequency heating of the solar wind triggered by low-frequency turbulence," Nature Astron. 6, 715 (2022)
- R. J. Ewart et al., "Relaxation to universal non-Maxwellian equilibria in a collisionless plasma," arXiv:2409.01742; see also A. Schekochihin's MMathPhys Lecture Notes, sec. 10-11
Nonthermal particle acceleration in extreme plasmas around black holes
Supervisors: Dmitri Uzdensky
Accreting supermassive black holes (SMBHs) residing in active galactic nuclei (AGN) at the centres of many galaxies, including the Milky Way, are arguably the most fascinating and enigmatic objects in the Universe. These black holes, and the powerful relativistic jets emanating from them, often exhibit spectacular, violent phenomena, such as bright high-energy flares with nonthermal X-ray and gamma-ray spectra. They are also the most likely generators of energetic non-electromagnetic observational "messengers": extremely relativistic cosmic rays and ultra-high-energy neutrinos. All these observational signals indicate that BH environments are very efficient relativistic-particle accelerators. Understanding how these cosmic accelerators work is an outstanding problem in high-energy astrophysics. Nonthermal particle acceleration is believed to be a natural product of nonlinear collective kinetic plasma processes, such as magnetic reconnection, shocks, and magnetised turbulence. How these processes operate under the extreme physical conditions in the exotic relativistic plasmas of accreting black holes is not well understood because the underlying physics is much richer than in more traditional plasmas (like ones in the solar system): special- and general-relativistic effects, strong interaction of the plasma with radiation, and quantum-electrodynamic effects such as pair creation all come into play in nontrivial ways. Elucidating the complex interplay of these effects with collective plasma processes and, consequently, their impact on particle acceleration and radiation presents an exciting theoretical challenge and is a vibrant frontier of modern plasma astrophysics research. This project will explore these fundamental plasma-theoretical questions and their observational implications through a combination of analytical and computational methods, with the balance between theory and computation to be decided based on the student's preferences.
Magnetic-field amplification by the fluctuation dynamo in astrophysical plasmas
Supervisors: Archie Bott, Alexander Shekochihin
Understanding cosmic magnetism is a long-standing problem in astrophysics. Astronomical observations of various astrophysical environments – for example, those of our own galaxy by the MeerKAT telescope [1] or of more distant galaxy clusters [2] – reveal the presence of finely structured magnetic fields of sufficient strength to affect the dynamics of such systems. A possible mechanism for explaining the presence and characteristics of these magnetic fields is the so-called fluctuation dynamo, whereby stochastic or turbulent motions of the plasma in which these magnetic fields are embedded amplify and maintain them [3]. The fluctuation dynamo has mostly been studied using simplified magnetohydrodynamic (MHD) plasma models whose key transport properties (viz., resistivity and viscosity) do not depend on macroscopic plasma properties such as density, temperature, and the magnetic field e.g., [4,5]. However, the plasma often found in the astrophysical systems of interest is often weakly collisional, and current theories for the transport properties of such plasma typically do predict a dependence on macroscopic plasma properties [6]. It is an outstanding question whether `anomalous’ transport properties can explain why certain predictions of the simplified theory of the fluctuation dynamo are at odds with astronomical observations (for example, that the energy-containing scale of the magnetic field is macroscopic). In this project, a student would explore how more realistic models of transport properties affect the fluctuation dynamo using both theory and numerical simulations, and then attempt to identify key differences with simplified dynamo theories that could be detected in astronomical observations.
Key reading
- I. Heywood et al., Nature 573, 235 (2019).
- C. L. Carilli & G.B. Taylor, Annu. Rev. Astron. Astrophys. 40, 319 (2002).
- F. Rincon, J. Plasma Phys. 85, 205850401 (2019).
- A. A. Schekochihin et al., Astrophys. J. 612, 276 (2004).
- A. K. Galishnikova, M.W. Kunz, A.A. Schekochihin, Phys. Rev. X 12, 041027 (2022)
- A. A. Schekochihin, S. C. Cowley, Phys. Plasmas 13, 056501 (2006).
Generating and sustaining radio filaments in astrophysical plasmas
Supervisors: Archie Bott, Alexander Shekochihin
One of the most intriguing findings from recent high-resolution astronomical observations of radio emission in astrophysical objects, such as galaxy clusters (as well as our own Galaxy), is the presence of collimated filaments of plasma [1-6]. These structures, which can extend from tens [2] to hundreds [4] of kiloparsecs, pose a significant theoretical challenge: how they are formed and persist are open questions. If the evolution of these plasma filaments were modelled accurately by hydrodynamics, they would quickly break up due to the Kelvin-Helmholtz and Rayleigh-Taylor instabilities. Yet they are there, and they shine with synchrotron radiation---which suggests that both the fluid instabilities are suppressed, and also that the filaments are capable of channelling high-energy cosmic rays, thought to produce the observed radio emission. A plausible hypothesis is that these filaments are threaded by magnetic fields with energy densities comparable to the thermal energy density of the plasma. However, the mechanism by which such strong magnetic fields could be generated in the low-density, high-temperature plasma typical of intergalactic space is a mystery. In this DPhil project, we will investigate how (or whether) strong compression and shearing of intergalactic plasma can lead to emergence of a "two-phase" intracluster medium: strongly and weakly magnetised, with a particular focus on the nonlinear evolution of the so-called mirror instability [7-9]. The research will involve analytical work, as well as fluid and kinetic.
Background Reading:
- R. J. van Weeran et al., Space Sci. Rev. 215, 16 (2019)
- M. Brienza et al., Nature Astron. 5, 1261 (2021)
- L. Rudnick et al., Astrophys. J. 935, 168 (2022)
- K. Rajpurohit et al., Astrophys. J. Lett. 927, L80 (2022)
- F. Yusef-Zadeh et al., Astrophys. J. Lett. 939, L21 (2022)
- E. Churazov et al., Astron. Astrophys. 686, A14 (2024)
- M. W. Kunz et al., Phys. Rev. Lett. 112, 205003 (2014)
- F. Rincon et al., MNRAS 447, L45 (2015)
- S. Melville et al., MNRAS 459, 2701 (2016)
Magnetised plasma turbulence: from laser lab to galaxy clusters
Supervisors: Gianluca Gregori, Archie Bott, Alexander Schekochihin
There are a number of possibilities within this project to design, take part in, and theorise about laboratory experiments employing laser-produced plasmas to model astrophysical phenomena and basic, fundamental physical processes in turbulent plasmas. Recent examples of our work in this field include turbulent generation of magnetic fields ("dynamo") [1,2], supersonic turbulence mimicking star-forming molecular clouds [3], diffusion and acceleration of particles by turbulence [4,5], suppression of thermal conduction in galaxy-cluster-like plasmas [6]. Our group has access to several laser facilities (including the National Ignition Facility, the largest laser system in the world). Students will also have access to a laser laboratory on campus, where initial experiments can be fielded. Depending on the student's inclinations, it is also possible to pursue a project focused on theory and/or numerical modelling of plasma phenomena in astrophysical and laboratory-astrophysical environments.
References:
- P. Tzeferacos et al., "Laboratory evidence of dynamo amplification of magnetic fields in a turbulent plasma," Nature Comm. 9, 591 (2018) (2019 APS Dawson Prize)
- A. F. A. Bott et al., "Time-resolved fast turbulent dynamo in a laser plasma," PNAS 118, e2015729118 (2021) (2020 EPS PhD Award in Plasma Physics)
- T. G. White et al.,"Supersonic plasma turbulence in the laboratory," Nature Comm. 10, 1758 (2019)
- A. F. A. Bott et al., "Proton imaging of stochastic magnetic fields," J. Plasma Phys. 83, 905830614 (2017)
- L. E. Chen et al., "Transport of high-energy charged particles through spatially intermittent turbulent magnetic fields," Astrophys. J. 892, 114 (2020)
- J. Meinecke et al., "Strong suppression of heat conduction in a laboratory analogue of galaxy-cluster turbulent plasma," Science Adv. 8, eabj6799 (2022)
Microphysics of Gamma-ray Bursts
Supervisors: Gianluca Gregori, Subir Sarkar, Dmitri Uzdensky
Gamma-ray bursts (GRBs) are among the most energetic events in the Universe. They occur at cosmological distances and are the result of the collapse of massive stars or neutron stars mergers, with emission of relativistic "fireballs" of electron-positron pairs. From astrophysical observations, a wealth of information has been gleaned about the mechanism that leads to such strong emission of radiation, with leading models predicting that this is due to the disruption of the beam as it blasts through the surrounding plasma. This produces shocks and hydromagnetic turbulence that generate synchrotron emission, potentially accelerating to ultra-high energies the protons which are observed on Earth as cosmic rays. However, there is no direct evidence of the generation of either magnetic fields or cosmic rays by GRBs. Estimates are often based on crude energy equipartition arguments or idealized numerical simulations that struggle to capture the extreme plasma conditions. We propose to address this lacuna by conducting laboratory experiments at accelerator facilities to mimic the jet propagation through its surrounding plasma. Our intial work [1] has demonstrated that we can create enormous beams of electron-positron plasmas that have properties very similar to GRB Fireballs. We are now planning new experiments at CERN as well as at Laboratori Nazionali Frascati (INFN, Italy) to fully characterize the interaction of these beams with a surrounding (ambient) plasma. Such experiments will enable in-situ measurement of the plasma properties, with exquisite details that cannot be achieved elsewhere. The experiments also complement numerical simulations by providing long measurement times extending into the non-linear regime where numerical simulations are not possible today. The proposed experiments will study fundamental physics processes, unveil the microphysics of GRBs, and provide a new window in high energy astrophysics using novel Earth-based laboratory tools.
Background Reading:
- C. D. Arrowsmith et al., "Laboratory realisation of relativistic pair-plasma beams," Nature Comm. 15, 5029 (2024)
Dynamic model of active galactic nuclei
Supervisors: Bence Kocsis
The recent discovery of gravitational waves opened new horizons for understanding the Universe. The measurements have unveiled an abundant population of stellar mass black hole mergers. The great challenge is to understand the possible astrophysical mechanisms that may lead to mergers. The existing theoretical models of their astrophysical origin are currently either highly incomplete or in tension with data (Barack+ 2018).
An interesting possibility is that stellar mass black hole mergers take place around supermassive black holes in the centers of galaxies. In these regions, the number density of stars and stellar black holes is up to a billion times higher than in the Solar neighborhood. Gas in the vicinity of a supermassive black hole collapses to a thin disc and rotates with nearly the speed of light, heats up, and releases a super-bright source of electromagnetic radiation. Such "quasars" or "active galactic nuclei" (AGN) often outshine all the stars of the host galaxy combined. However, a growing number of observations are in tension with current models of AGN (Antonucci 2015).
In this project, the student will work with Prof. Bence Kocsis to build a comprehensive model of AGN accounting for the population of stars and stellar mass black holes which surround this region. The objects in the disc twist and warp the disc gravitationally and contribute to heating the disc. The gas in turn slows down the objects, and causes them to settle into the disc, and catalyzes the formation of binaries, ultimately leading to mergers. We study the evolution of these systems, determine the rate of black hole mergers in these environments, and examine the distinguishing features of electromagnetic and gravitational waves emitted by this source population.
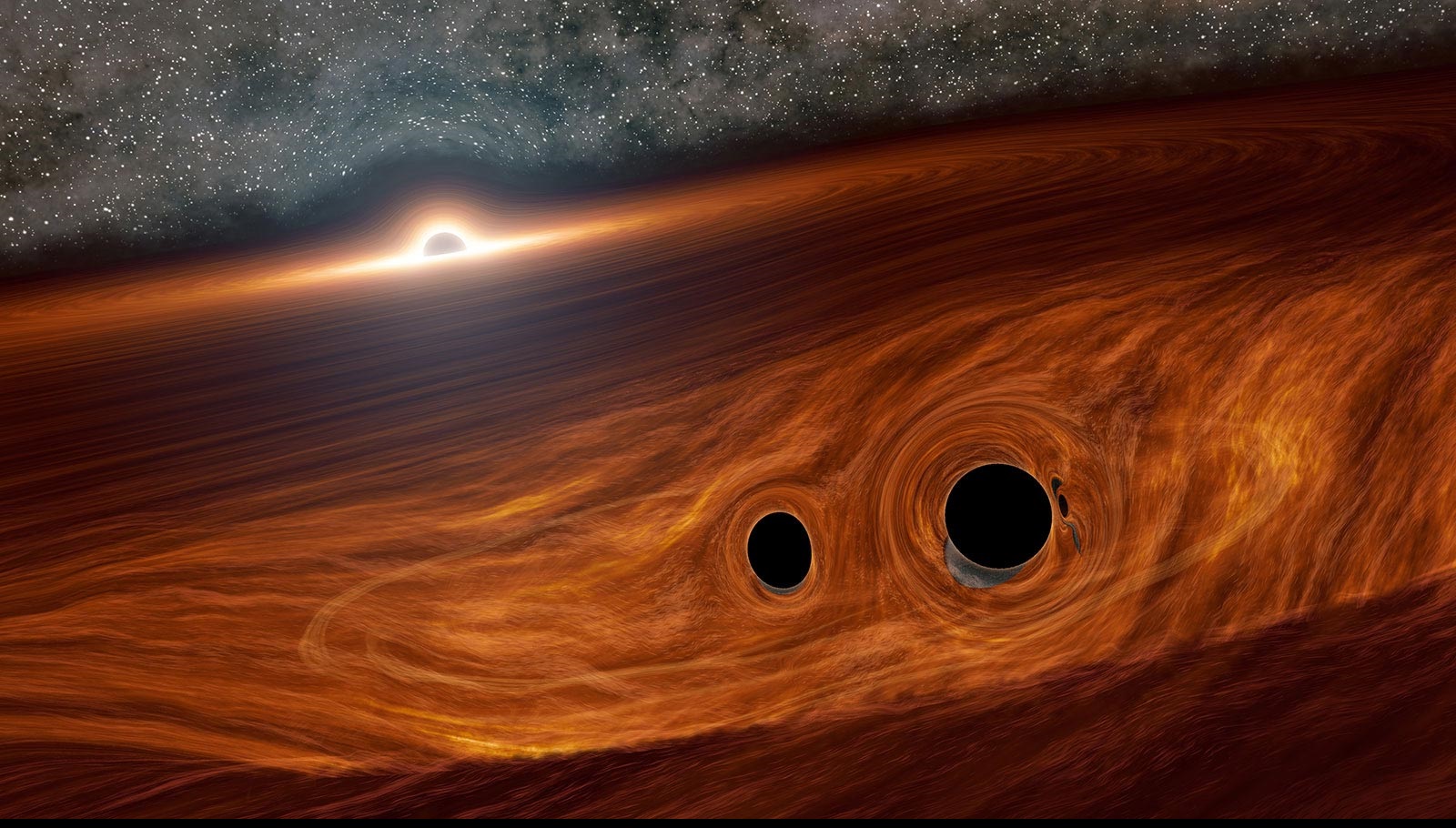
Links to further reading:
- Antonucci R., 2015, eprint arXiv:1501.02001
- LIGO, VIRGO, KAGRA Scientific Collaboration, 2023, PRX, 13, 041039
Link: https://ui.adsabs.harvard.edu/abs/2023PhRvX..13d1039A/abstract - Barack L. et al., 2019, Classical and Quantum Gravity, Volume 36, Issue 14, article id. 143001
Link: https://arxiv.org/abs/1806.05195 - Bartos, Kocsis, Haiman, Marka, 2017, ApJ, 835, 165
Link: https://ui.adsabs.harvard.edu/abs/2017ApJ...835..165B/abstract - Tagawa, Haiman, Kocsis, 2020, ApJ, 898, 25
Link: https://arxiv.org/abs/1912.08218
Waves and wobbles in galactic discs
Supervisors: John Magorrian
The stellar and gaseous discs are subject to a host of poorly understood dynamical phenomena, from obvious features such as bars and spiral arms to more subtle ones such as warps and phase spirals. The most immediate way of developing insight into these processes is by using N-body simulations, which, although superficially simple, take skill and insight both to set up and to analyse. We have recently developed practical schemes for applying the methods of linear response theory as an alternative to N-body methods for investigating how discs respond to perturbations, finding excellent agreement for small perturbations of razor-thin discs. Two open questions: (i) how best in practice to extend this formalism to deal with fully three-dimensional discs? (ii) how to couple this machinery (either 2d or 3d) to observations of our own Galactic disc to infer its present state and recent history?
Further reading