Below is a list of DPhil projects within the Atomic and Laser Physics sub-department; potential graduate students are encouraged to contact supervisors if they have any questions with regard to these projects.
Quantum computing with trapped ions
Trapped-ion devices have demonstrated, on a small number of qubits, all the building-blocks required to build a quantum computer with precision better than any competing technology. The aim of this project is to develop and utilise a world-class intermediate-scale quantum computer that, by virtue of high-fidelity any-qubit-to-any-qubit entangling gates along with low error rates, will operate at a performance level currently unachievable in any other architecture.
This is a challenging project which will push the limits of laser technology, quantum/classical control techniques, and quantum algorithm design.
The project will involve both experimental and theoretical work, including:
- building an apparatus that uses a newly developed type of trapped-ion qubit
- obtaining precision coherent control over individual atomic ions
- developing and applying new theoretical tools to understand and optimise many-qubit couplings
For further details https://www.physics.ox.ac.uk/research/group/ion-trap-quantum-computing/research-areas/abaqus
Fast, high-fidelity entanglement via optical phase control
Trapped-ion devices have demonstrated, on a small number of qubits, all the building-blocks required to build a quantum computer with precision better than any competing technology. However the speed of these devices, limited by the entangling gates, has not increased commensurately. The aim of this project is to change this by exploiting optical phase control to significantly speed up trapped-ion entangling gates whilst also removing several currently limiting fundamental sources of error.
In preliminary work, we have recently demonstrated the first high-speed entangling logic gates for trapped-ion qubits [Schafer et al., Nature 555, 75 (2018)]. We achieved a fidelity of 99.8% for a 1.6µs gate time, close to the highest reported two-qubit gate fidelities of 99.9%, but more than an order of magnitude faster. Over the course of this project we will extend this proof-of-concept technique to demonstrate the first high-speed control of multi-qubit registers.
The project will involve both experimental and theoretical work, including:
- developing and numerically modeling phase-controlled fast entangling gate dynamics
- building a new apparatus optimised for high-speed multi-qubit entangling gates
- sophisticated classical control techniques to precisely control the optical interaction phase of multi-qubit register
For further details https://www.physics.ox.ac.uk/research/group/ion-trap-quantum-computing/research-areas/fast-gates
Breaking symmetry with light: ultra-fast ferroelectricity and magnetism from non-linear phononics
Professor Andrea Cavalleri and Professor Paolo Radaelli
A collaboration between Prof. Paolo G. Radaelli and Prof. Andrea Cavalleri, who holds a joint appointment between the Clarendon Laboratory and the Max Planck Institute for the Structure and Dynamics of Matter, (Hamburg).
The use of light to control the structural, electronic and magnetic properties of solids is emerging as one of the most exciting areas of condensed matter physics. One promising field of research, known as femto-magnetism, has developed from the early demonstration that magnetic ‘bits’ in certain materials can be ‘written’ at ultra-fast speeds with light in the visible or IR range [1]. More radically, it has been shown that fundamental materials properties such as superconductivity can be ‘switched on’ transiently under intense illumination [2]. Recently, the possibilities of manipulating materials by light have been greatly expanded by the demonstration of mode-selective optical control, whereby pumping a single infrared-active phonon mode results in a structural/electronic distortion along the coordinates of a second, anharmonically coupled Raman mode – a mechanism that was termed ‘nonlinear phononics’. Crucially, the Raman distortion is partially rectified, meaning that it oscillates around a different equilibrium position than in the absence of illumination. Very recently, it was realised that, under appropriate conditions, the rectified Raman distortion can transiently break the structural and/or magnetic symmetry of the crystal. Such symmetry breaking persist for a time corresponding to the carrier envelope of the pump, which can be less than a picosecond, and can give rise to the ultra-fast emergence of ferroic properties such as ferromagnetism and ferroelectricity. Through symmetry analysis and first-principle calculations, we have identified several promising ‘photo-ferroic’ materials that should display these effects, with potential applications in ultra-low-power information storage, ultra-fast electronics and many more.
This DPhil project will give the successful candidate the opportunity to pioneer this new field of research. Initial experiments on the candidate ‘photo-ferroic’ materials that we have already identified will be performed at the Max Planck Institute for the Structure and Dynamics of Matter in Hamburg, Germany. As a mode-selective pump, we will employ coherent laser radiation in the THz or far-IR range with sub-ps carrier envelopes, while the transient emergence of the ferroic properties will be probed with second-harmonic generation (SHG) and/or Faraday rotation of near-infra-red light. Later on in the project, changes in the crystal and magnetic structures of the materials will be probed with X-rays at free electron laser sources such as the European XFEL in Hamburg. Meanwhile, the candidate will develop search strategies for new classes of ‘photo-ferroic’ materials, based on symmetry and density functional theory calculations. He/she will develop the materials specifications in collaborations with crystal growers in Oxford and elsewhere, and will be involved hands on in all aspects of the design and realisation of the experiments and the data analysis.
The experimental part of this project will be predominantly based in Hamburg, so it is essential for the candidate to be willing and able to be based in Germany for extended periods during the DPhil.
[1] Femtomagnetism: Magnetism in step with light. Uwe Bovensiepen, Nature Physics 5, 461 - 463 (2009)
[2] See for example M. Mitrano,et al., ‘Possible light-induced superconductivity in K3C60 at high temperature’, Nature, 530, 461–464 (2016)
[3] Nonlinear phononics as an ultrafast route to lattice control, M. Först et. al., Nature Physics, 7, 854–856 (2011)
Experiments with ultracold atoms and Bose-Einstein condensates
Professor Foot’s research group investigates the fascinating properties on ultracold quantum gases at temperatures of a few tens of nanokelvin. Samples are produced using laser light to cool atoms from room temperature and then confining them in a magnetic trap where evaporation leads to further cooling. These processes increase the phase-space density by many orders of magnitude to reach quantum degeneracy (Bose-Einstein condensate for atoms with integer spin). The research group in Oxford has pioneered new methods of trapping ultracold atoms using a combination of radiofrequency and static magnetic fields that is a very powerful tool for probing 2D quantum systems. The latest results have been reported in a very recent paper on the Universal scaling of the dynamic BKT transition in quenched 2D Bose gases, Science 382:6669 (2023) 443.
Research on `Investigating non-equilibrium physics and universality using two-dimensional quantum gases’ is funded by a new EPSRC grant [1]. Coherent splitting and matter-wave interference techniques enable comprehensive read out the quantum information from these systems to study fundamental questions such as how an isolated quantum system evolves towards equilibrium (or quasi-equilibrium states). We are working with theoretical colleagues to make detailed comparison with quantum statistical mechanics. Understanding the fundamental quantum physics of many-body system has important implications for quantum devices and technology based on them.
Future research directions include experiments on weak quantum measurements (sometimes called quantum non-demolition) on atoms in double-well potentials (bosonic Josephson junctions), squeezed states of the atoms and extensions to quantum gases that are a mixture of multiple species such a rubidium and strontium atoms.
[1] https://gow.epsrc.ukri.org/NGBOViewGrant.aspx?GrantRef=EP/S013105/1
Optical lattice clocks for fundamental physics and redefinition of the second
This project has been filled for 2021/22.
This is an experimental project, working with some of the UK’s best atomic clocks at the National Physical Laboratory. The clocks are based on strontium atoms, which are laser-cooled to a temperature of 1 µK, and then trapped in an optical-lattice dipole trap. The trapped atoms are probed using an ultra-stable clock laser, which is tuned in frequency to address a narrow optical transition at 429 THz. By measuring whether the clock laser excites the atoms or not, we can steer the laser to “tick” at a rate matching the narrow atomic transition frequency. Following this procedure, the clock laser can measure the passage of time to 18 digits of precision – enough to resolve the gravitational redshift from a change in height of just a few cm on the surface of the earth.
Already optical lattice clocks reach fractional frequency uncertainties and instabilities more than 100 times lower than the best caesium primary frequency standards. As a result, optical lattice clocks are a likely candidate for a future redefinition of the SI second. However, before such a redefinition, it must be shown that these systems can be engineered to run reliably, and that frequencies derived from such clocks are reproducible. We achieve this through real-time comparison of NPL’s clocks with others across Europe via optical fibre links, and across the globe via satellites including the soon-to-be-operational Atomic Clock Ensemble in Space (ACES). These ultra-precise clock-clock comparisons also provide valuable insight into many open problems in fundamental physics, underpinning the hunt for dark matter, tests for violations of relativity, and constraints on possible variations in fundamental constants. To extend the reach of clocks towards new applications, new techniques must be developed to overcome the current limitations on clock performance. This effort will be the focus of the current PhD placement, for instance by exploring how quantum entanglement can be leveraged to supress frequency instability arising from quantum projection noise by engineering ‘spin-squeezed’ states.
Development of Ultra-Sensitive Detectors for High-Energy Density Plasma Experiments
Dr. Boon Kok Tan and Professor Gianluca Gregori
Since the invention of the chirped pulse amplification technique by Strickland and Mourou (awarded the 2018 Nobel Prize in Physics), high-intensity lasers focused on solid foils have enabled the acceleration of electrons to relativistic velocities through powerful electric fields. These electrons then interact with nuclei, producing abundant jets of electron-positron pairs. These jets, which mimic the properties of gamma-ray fireballs, provide a means to study the microphysics of extreme astrophysical phenomena, such as those occurring near the event horizon of black holes, while also serving as valuable tools for fundamental physics investigations.
The goal of this project is to develop a novel detector using superconducting quantum technologies to study the secondary emission from the high-energy gamma-ray collisions during pair production. By doing so, we aim to optimise jet emission and characterise its properties. The developed detectors can also be used to detect non-astronomical sources, such as lab-based radiometry, provided they operate within the designated mass range (several hundred GHz to a few THz).
Several promising candidates exist for developing such superconducting quantum detectors. For this project, we will explore the use of Superconducting Tunnel Junctions (STJs) and/or Superconducting On-chip Fourier Transform Spectrometers (SOFTS) with Kinetic Inductance Detector (KID) technologies. STJs have been the primary detectors for astronomy below 1 THz, while KIDs have been used to detect photons ranging from the sub-millimetre to the X-ray regime. In this project, the student will first assess the feasibility of using one of these technologies for detecting the secondary high-energy particles with high resolution. Once the most suitable technology is identified, the student will design and fabricate the devices, and set up the experimental arrangement needed to test the detector’s performance in a real-world setting, such as a large synchrotron laser emission facility in Italy.
This programme consists of two complementary scientific objectives: first, the development of superconducting quantum detectors, and second, the use of these detectors to investigate the microphysics of extreme astrophysical phenomena in laboratory-based settings. The student will also have the opportunity to participate in device fabrication using state-of-the-art cleanroom facilities, either at Oxford or in collaboration with the Paris Observatory or IRAM in France.
This project is well-suited to a student with an interest in theoretical quantum sensors, superconducting electromagnetism, and high-energy astrophysics, who also enjoys coding, lab-based experimental work, and data analysis. Our cryogenic detector laboratory is equipped with sub-Kelvin dilution refrigerators and advanced test and measurement equipment. The student will receive support from technicians in lab, postdocs, and supervisors, and will have access to both commercial and custom software and code for their research.
Background Reading:
- https://www.nature.com/articles/s41467-024-49346-2
- https://journals.aps.org/prl/abstract/10.1103/PhysRevLett.105.015003
- https://www.spiedigitallibrary.org/conference-proceedings-of-spie/8453/84532N/High-resolution-gamma-ray-detection-using-phonon-mediated-detectors/10.1117/12.926829.full?SSO=1
- https://iopscience.iop.org/article/10.1088/1361-6668/acca51/pdf
- https://link.springer.com/article/10.1007/s10909-020-02490-7
- https://www.sciencedirect.com/science/article/pii/S0921453417300643
Related Images:
Microphysics of Gamma-ray Bursts
Professor Gianluca Gregori Prof Subir Sarkar and Prof Dmitri Uzdensky
Gamma-ray bursts (GRBs) are among the most energetic events in the Universe. They occur at cosmological distances and are the result of the collapse of massive stars or neutron stars mergers, with emission of relativistic "fireballs" of electron-positron pairs. From astrophysical observations, a wealth of information has been gleaned about the mechanism that leads to such strong emission of radiation, with leading models predicting that this is due to the disruption of the beam as it blasts through the surrounding plasma. This produces shocks and hydromagnetic turbulence that generate synchrotron emission, potentially accelerating to ultra-high energies the protons which are observed on Earth as cosmic rays. However, there is no direct evidence of the generation of either magnetic fields or cosmic rays by GRBs. Estimates are often based on crude energy equipartition arguments or idealized numerical simulations that struggle to capture the extreme plasma conditions. We propose to address this lacuna by conducting laboratory experiments at accelerator facilities to mimic the jet propagation through its surrounding plasma. Our intial work [1] has demonstrated that we can create enormous beams of electron-positron plasmas that have properties very similar to GRB Fireballs. We are now planning new experiments at CERN as well as at Laboratori Nazionali Frascati (INFN, Italy) to fully characterize the interaction of these beams with a surrounding (ambient) plasma. Such experiments will enable in-situ measurement of the plasma properties, with exquisite details that cannot be achieved elsewhere. The experiments also complement numerical simulations by providing long measurement times extending into the non-linear regime where numerical simulations are not possible today. The proposed experiments will study fundamental physics processes, unveil the microphysics of GRBs, and provide a new window in high energy astrophysics using novel Earth-based laboratory tools.
Background Reading:
1. C. D. Arrowsmith et al., "Laboratory realisation of relativistic pair-plasma beams," Nature Comm. 15, 5029 (2024)
Related Images:
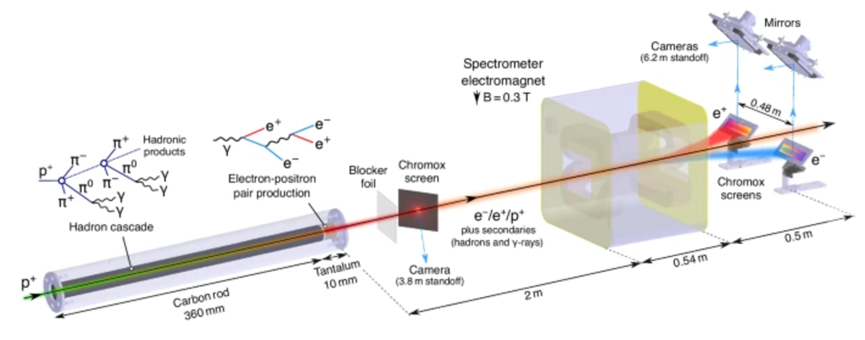
Magnetised plasma turbulence: from laser lab to galaxy clusters
Professor Gianluca Gregori, Dr Archie Bott, and Prof Alexander Schekochihin
There are a number of possibilities within this project to design, take part in, and theorise about laboratory experiments employing laser-produced plasmas to model astrophysical phenomena and basic, fundamental physical processes in turbulent plasmas. Recent examples of our work in this field include turbulent generation of magnetic fields ("dynamo") [1,2], supersonic turbulence mimicking star-forming molecular clouds [3], diffusion and acceleration of particles by turbulence [4,5], suppression of thermal conduction in galaxy-cluster-like plasmas [6], production of pair-plasma jets [7]. Our group has access to several facilities (including the National Ignition Facility, the largest laser system in the world). Students will also have access to a laser laboratory on campus, where initial experiments can be fielded. Depending on the student's inclinations, it is also possible to pursue a project focused on theory and/or numerical modelling of plasma phenomena in astrophysical and laboratory-astrophysical environments.
Background Reading:
1. P. Tzeferacos et al., "Laboratory evidence of dynamo amplification of magnetic fields in a turbulent plasma," Nature Comm. 9, 591 (2018)
2. A. F. A. Bott et al., "Time-resolved fast turbulent dynamo in a laser plasma," PNAS 118, e2015729118 (2021)
3. T. G. White et al.,"Supersonic plasma turbulence in the laboratory," Nature Comm. 10, 1758 (2019)
4. A. F. A. Bott et al., "Proton imaging of stochastic magnetic fields," J. Plasma Phys. 83, 905830614 (2017)
5. L. E. Chen et al., "Transport of high-energy charged particles through spatially intermittent turbulent magnetic fields," Astrophys. J. 892, 114 (2020)
6. J. Meinecke et al., "Strong suppression of heat conduction in a laboratory analogue of galaxy-cluster turbulent plasma," Science Adv. 8, eabj6799 (2022)
Extreme physics with high power lasers
Prof. Gianluca Gregori, Prof. Subir Sarkar and Prof. Bob Bingham (STFC)
It is well known that particle-production phenomena can occur in a curved or dynamic spacetime. For example, thermal radiation can arise from particle production near the event horizon of a black hole, an effect commonly known as the Hawking radiation. Thanks to the development of high-intensity lasers, and by virtue of the equivalence principle, an electron placed at the focus of such beams will experience an acceleration comparable to what it would feel if placed near the event horizon of a ~10−12 Msun black hole. Indeed for such low mass black holes the surface gravity is strong enough that pairs of entangled photons can be produced, with one escaping to infinity. While the scientific community generally believes that the derivation of Unruh/Hawking radiation is sound, this is nevertheless made possible by several approximations that have not been tested. An experimental test of the Hawking-Unruh radiation will the very important to further our understanding of fundamental quantum gravity processes. Moreover, the ideas here can be easily generalized, as the accelerated electron can couple not only with photons but also with the emission of other particles, possibly beyond the standard model, such as gravitons, neutrinos, axions, dark photons, or millicharged particles. The ideal candidate is expected to develop the theory underlying these experiments, defining the required experimental parameters and the proposal for possible experiment at high power laser facilities.
Background Reading:
Measuring Temperatures at Planetary Core Conditions
Professor Sam Vinko and Professor Justin Wark
Signification progress has been made over the past few years in techniques to compress matter on nanosecond timescales, using laser ablation, to pressures comparable to those at the centre of planets. It is known that the matter can be kept cool enough to remain solid, as we can do femtosecond x-ray diffraction (from the output of x-ray lasers) to look at the lattice during the compression process - with diffraction data giving the density. Pressures can be measured with sophisticated measurements of the effect sound speed in the material, but a long-standing problem is how to measure temperature accurately on such ultra short timescales.
This DPhil project, which will be a mix of theory (simulation) and experiment, will attempt to address that issue by evaluating several proof of principle concepts for such a measurement. Simulations will be performed at Oxford, but the experiments will require visits to the world’s x-ray lasers at Stanford in the US, and Hamburg in Germany. The project would suit someone interested in both theory and experiment, and has interests in generating in the laboratory the sort of conditions that only exist elsewhere in the Universse.
This studentship will be supported by the Oxford Centre for High Energy Density Science (OxCHEDS); thanks to OxCHEDS’ industrial partnership with AWE, it will be fully funded for UK students with a significantly supplemented DPhil stipend.
Further details can be provided upon request.
Relevant References
[1] Wark, McMahon, Eggert, Femtosecond Diffraction and Dynamic High Pressure Science, J. Appl. Phys. 132, 080902 (2022)
Plasma accelerators
In a laser wakefield accelerator an intense laser pulse propagating through a plasma excites a trailing plasma wave via the action of the ponderomotive force, which acts to expel electrons from the region of the laser pulse. The longitudinal electric field in this plasma wakefield can be as high as 100 GV / m, more than three orders of magnitude larger than that found in conventional RF accelerators such as those used at CERN. Particles injected into the correct phase of the plasma wave can therefore be accelerated to energies of order 1 GeV in only a few tens of millimetres. Laser-driven plasma accelerators could therefore drive novel, very compact sources of particles and ultrafast radiation.
Theoretical and experimental work on plasma accelerators in Oxford is undertaken by a collaboration of research groups in the sub-departments of Particle Physics and Atomic & Laser Physics. For this reason applications to work in this area should be made to the sub-departments of Atomic & Laser physics AND to Particle Physics.
Our work in this area is undertaken in the Oxford Plasma Accelerator Laboratory (OPAL), and at national laser facilities in the UK and elsewhere. Further information on our research can be found on the laser-plasma accelerator group website.
We are offering three DPhil projects to start in October 2025, as outlined below.
1. Controlled electron injection in plasma-modulated plasma accelerators (P-MoPA)
Most work to date on laser-driven plasma accelerators has been done with single driving pulses, which must have an energy of order 1 J and a duration shorter than the plasma period (around 100 fs). These demanding parameters can be generated by Ti:sapphire laser systems, but these lasers have very low efficiencies (< 0.1%) and (for joule-scale pulse energies) are limited to pulse repetition rates below 10 Hz. These repetition rates and efficiencies are too low for the most demanding applications, such as driving compact light sources.
We recently proposed that GeV-scale plasma accelerators could be driven by commercially available thin-disk lasers (TDLs). These systems can provide the required pulse energy at pulse repetition rates in the kilohertz range – but the pulses that they generate are too long (a few picoseconds) to drive a plasma wave directly. We have developed a new concept to circumvent this: the Plasma-Modulated Plasma Accelerator (P-MoPA).
In a P-MoPA, a long (~ 1 ps), high-energy (~ 1 J) TDL pulse is modulated spectrally by co-propagating it in a HOFI channel with a low-amplitude plasma wave driven by a short (< 100 fs), low-energy (< 100 mJ) “seed” laser pulse. This causes the drive pulse to develop spectral sidebands spaced by the plasma frequency. Removing the spectral phase of the sidebands in an optical “compressor” converts the spectral modulation to a temporal one, thereby forming a train of short pulses spaced by the plasma period. This pulse train can then resonantly excite a high-amplitude plasma wave in an accelerator stage with a plasma density equal to that of the modulator plasma.
In order to accelerate electrons in the accelerator stage of the P-MoPA, it will be necessary to develop novel methods for injecting a high-quality electron bunch into the resonantly excited, quasi-linear wakefield driven in the accelerator stage. The novel architecture of the P-MoPA scheme means that current schemes for controlling electron injection into wakefields driven by single laser pulses may not work well. In this project the student will explore possible injection schemes using particle-in-cell codes and other numerical tools. This will be accompanied by experimental work in Oxford and at international high-power laser facilities. It may be necessary for the student to work for one or more extended periods with our colleagues at Ludwig-Maximilians Universität, Munich.
2. Radiation generation in high-repetition rate plasma accelerators
Laser-driven plasma accelerators are ideally suited to driving very compact X-ray sources, with many potential applications in science and medicine, such as high-resolution medical imaging of deep-seated tumours.
Radiation can be generated from a laser-accelerated electron bunch in several ways. The transverse electric fields within the plasma wave itself can cause the electron bunch to oscillate as it is accelerated. This leads to the generation of broad-band “betatron” radiation, with photon energies typically in the 10 – 30 keV range. Alternatively, colliding a laser-accelerated bunch with an intense, counter-propagating laser pulse can generate Thomson and Compton radiation with photon energies in the 100 keV – 1 MeV range.
In this project the student will explore methods for generating X-rays from laser-accelerated electrons. Of particular interest will be radiation generation from electron bunches accelerated in HOFI channels by single- or multiple laser pulses (e.g. in a P-MoPA). It is anticipated that the project will involve a mix of numerical simulations and experiments in Oxford at OPAL and at national and international high-power laser facilities such as the Extreme Photonics Application Centre (EPAC) at Harwell..
3. Energy recovery in plasma accelerators
Laser- and particle-driven plasma accelerators have the potential to drive future generations of particle colliders. However, in order to detect and study rare events the collider must have a high luminosity, which requires accelerating bunches of high charge, to high energies, at high mean repetition rates. The time-averaged power of the laser- or particle-driver in future colliders is expected to be of order 100s kW per acceleration stage. As a consequence the accelerator stage must operate with high efficiency since otherwise: (i) unused wakefield energy damage the structure containing the plasma; and (ii) the running costs would be prohibitive.
A potential solution to this problem is to use a trailing laser pulses to damp the plasma wakefield remaining after the acceleration event. If the trailing pulse is out of resonance with the wakefield, then the frequency of the laser pulse will be up-shifted, allowing the unused wakefield energy to be removed in the form of blue-shifted photons. In principle the up-shifted laser pulse can be used elsewhere in the accelerator, or its energy can be re-converted to electrical power.
This project will involve particle-in-cell simulations in order to devise promising strategies for this energy recovery concept. Experiments will then be undertaken in Oxford at OPAL — and potentially at national or international laboratories, such as the Extreme Photonics Application Centre (EPAC) at Harwell.
Demonstration of a cavity-based photonic entangler
Entanglement, in which two quantum systems can exhibit correlations that are greater than the limit allowed by classical physics, is one of the most intriguing predictions of quantum mechanics. Entanglement between remote atoms or ions is a key resource for quantum computing, and plays a central role in NQIT, the Oxford-led quantum technology hub.
We propose two schemes for entangling remote atoms: one probabilistic and one deterministic. In the probabilistic scheme, two distant atoms each emit a photon which are combined at the two input ports of a 50:50 beamsplitter. If the photons are detected at different output ports, then the atoms are projected into an entangled state. In place of a simple beam splitter, we also anticipate using more complex photonic networks [A. Holleczek, PRL 117, 023602 (2016)] in combination with active optical photon switching and routing. In the deterministic scheme, an atom emits a single photon which is reabsorbed by a second atom by running the emission process in reverse [J. Dilley, PRA 85, 023834 (2012)]. In doing so, the state of the first atom is entangled with that of the second. In both schemes, a high-finesse optical cavity is used to enhance the light-atom interactions.
Currently, we have two optical cavity experiments with random atom loading. The first phase of the project will be to build an optical dipole trap to permanently hold single atoms in the cavities. The feasibility of this approach has recently been demonstrated [D. Stuart, arXiv:1708.06672], and suitable fibre-tip and FIB-milled cavity mirrors are at present under development. The second phase will be to generate and quantify the entanglement between the two remote atoms using full Bell-state tomography.
Person specification
This is a highly challenging experimental project which will push the limits of laser and optical technology. It would suit a student with experience in atomic and laser physics and a keen interest in exploring quantum phenomena experimentally.
Work environment
The research team does encompass two postdocs and four graduate students which operate three laboratories dedicated to cavity-qed and atom-photon coupling in cavities at the Physics department of the University of Oxford. The work space is well equipped, comprising four vacuum chambers for studying atom-photon coupling in cavities, a large number of ECDL and fibre lasers for atom manipulation, a frequency comb for synchronously stabilising all laser and cavity frequencies, and a large battery of single-photon counters. The project builds on the current work by other graduate students in our group, atom-cavity coupling and strong cavity coupling.
The new student will directly contribute towards achieving cavity-mediated remote entanglement. All necessary apparatus exists within NQIT, including high-finesse cavities, vacuum chambers, and all lasers for trapping and driving the photon production process. Close support on a day-to-day basis will be provided by at least one Oxford PDRA for the duration of the project.
Machine Learning for Optimum Control in Quantum Technology
We use machine learning to control physical systems at the single-quantum level, which is a prerequisite for large-scale quantum computing. The method applies to many quantum-processing platforms, such as quantum networks coupling atoms or ions, cavity-based schemes, rf-pulse controlled superconductive circuits, and other systems performing quantum operations controlled by suitably shaped pulses. Classical computing techniques improve operations [1], and make larger problems accessible with state-of-the-art hardware. We have shown that optimised pulses produce photons from atomic systems with high efficiency and repetition rates [2], and we applied reinforcement learning to control the quantum dynamics of atomic systems [3].
This project expands this research by developing a universal reinforcement-learning based method applicable to a large variety of systems, including superconductive quantum devices. Our goal is to extend quantum control techniques with machine learning, leveraging the unique experience in our group to tackle specific challenges of superconducting hardware and coherent population transfer to realise an efficient three-qubit gate. The three major milestones are:
- Enhanced Device Characterisation: Implementation of machine learning techniques to analyse and improve the modelling, including device imperfections, based on data of our own atom-cavity systems and of accessible superconducting quantum devices.
- Optimisation of Control Signals: Apply reinforcement learning techniques to optimise control pulse sequences through numerical simulations, with the ultimate goal of realising a Toffoli gate. This extends coherent population transfer techniques which has we pioneered for atomic systems.
- Testing and Validation of the optimised pulse sequences using a combination of digital twin simulations and physical device testing, both on superconducting devices accessible to us via the NQCC, and with atomic systems in our own laboratories.
Improving multi-qubit control is crucial for scaling quantum computing hardware. An optimised pulse sequence for three-qubit gate operations would significantly reduce the computational overhead and thus extend the reach of current hardware capabilities.
The project is based on our own activities, and we anticipate a collaboration with the NQCC to test and validate other platforms than our own atom-cavity systems. With the project making extensive use of machine and reinforcement learning, it is also open to applicants with a suitable background in computer science, not only for physicists. If necessary, supplementary training in quantum technologies might be provided.
[1] M Krenn, J Landgraf, T Foesel, and F Marquardt, Phys. Rev. A 107, 010101 (2023)
[2] Jan Ole Ernst et al, J. Phys. B: At. Mol. Opt. Phys. 56 205003 (2023)
[3] Jan Ole Ernst et al, Scalable Quantum Control via Physics Constrained Reinforcement Learning
https://openreview.net/forum?id=YPvI7SofeZ (ICLR Conference Submission)
Superresolution imaging via linear optics in the far-field regime
Diffraction limits the resolution of imaging devices, such as microscopes and telescopes. Enhancing the resolution beyond this limit has been a crucial outstanding problem for many years. A number of solutions have been realized; however, all of them so far relied either on near-field or nonlinear-optical probing, which makes them invasive, expensive and not universally applicable. It would therefore be desirable to find an imaging technique that is both linear-optical and operational in the far-field regime. A recent theoretical breakthrough demonstrated that “Rayleigh’s curse” can be resolved by coherent detection of the image in a basis of certain transverse electromagnetic modes. This method offers a significant improvement with respect to the traditional imaging procedure, which consists in measuring the incoherent intensity distribution over the image plane. To date, there exist proof-of-principle experimental results demonstrating the plausibility of this approach. The objective of the project is to test this approach in a variety of settings that are relevant for practical application, evaluate its advantages and limitations and construct a linear optical microscope whose resolution exceeds the state of the art. If successful, it will result in a revolutionary imaging technology with a potential to change the faces of all fields of science and technology that involve optical imaging, including astronomy, biology, medicine and nanotechnology, as well as optomechanical industry.
The group website can be found here: quantum and optical technology group
Optical neural networks
Machine learning has made enormous progress during recent years, entering almost all spheres of technology, economy and our everyday life. Machines perform comparably to, or even surpass humans in playing board and computer games, driving cars, recognizing images, reading and comprehension. It is probably fair to say that an artificial neural network can perform better than a human in any environment it has complete knowledge of. These developments however impose growing demand on our computing capabilities, including both the size of neural networks and the processing rate. This is particularly concerning in view of the decline of Moore’s law.
The project is to implement artificial neural networks using optics rather than electronics. Optical neural networks would enable us to enhance both the power efficiency and speed of neural networks by several orders of magnitude. The specific aim is to develop a conceptually novel deep optics neural network system for computer vision. This system will allow an optical neural network to “see” and interpret objects directly, bypassing the processing bottleneck associate with converting an image into an electronic form. Such a system will have ultra-low latency and find applications in autonomous vehicles, remote sensing and intelligent robotics.
The group website can be found here: quantum and optical technology group
Coherent Ising machines
An important class of computational problems is combinatorial optimisation: finding an optimal solution among a discrete set. Combinatorial optimisation problems are NP hard, meaning that they rapidly become intractable with the increasing problem sizes. Yet they have an enormous range of application, ranging from logistics and investment portfolio optimization to the modelling of protein molecule folding and quantum condensed matter physics. In this project, we construct a coherent Ising machine – a network of quantum optical parametric oscillators that are based on optical nonlinearity in atomic vapour and are coupled by means of a spatial light modulator. When launched, this system “anneals” itself to a phase state encoding the solution to a given quadratic unconstrained binary optimization problem.
The group website can be found here: quantum and optical technology group
Laser-plasma interaction physics for inertial fusion and extreme field science
Intense lasers have extraordinary properties. They can deliver enormous energy densities to target, creating states of matter in the laboratory that are otherwise only found in exotic astrophysical phenomena, such as the interiors of stars and planets, the atmospheres of white dwarfs and neutron stars, and in supernovae. The behaviour of matter under these extreme conditions of density and temperature is a fascinating area of study, not only for understanding of fundamental processes that are, in most cases, highly non-linear and often turbulent, but also for their potential applications for other areas of the natural sciences.
My team is working on:
- Inertial fusion - applications include fundamental studies for energy generation and the transition to a carbon-free economy and the development of the brightest possible thermal source for neutron scattering science
- New high power optical lasers and coherent X-ray using non-linear optical properties in plasma
- Novel particle accelerators via laser and beam-driven wakefields - including the AWAKE project at CERN, as a potential route for a TeV e-e+ collider
- New approaches to hyperspectral imaging, supported by my group’s spin-out company Living Optic
The understanding of these physical processes requires a combination of observation, experiment and high performance computing models on the latest supercomputers. My team is versatile, combining experiment, theory and computational modelling, including applications of machine learning - I can offer projects in all of these areas More specifically:
Project 1. I have been awarded an UKRI-STFC grant to implement a new optical diagnostic on the AWAKE run II experiment at the CERN laboratory, in conjunction with the John Adams Institute. The aim is the visualise plasma wakefields as they evolve in the 10-metre long plasma column. This will be the first time that the structure of a beam-driven wakefield accelerator will be measured in the laboratory and promises exciting discoveries of the real structure of wakefields generated by the Super Proton Synchrotron beam (operating at 400 GeV). The student will help with the design and implementation of the oblique angle frequency domain holographic set-up, visualise the outcome and compare the data with state-of-the-art computer simulations.
Project 2. I have submitted a large collaborative Synergy grant application (with Prof Luis Silva and Prof Mattias Marklund) to the European Research Council on photon-photon scattering using intense laser pulses. The idea is to polarise the vacuum (which comprises virtual electron-positron pairs) using three ultra-intense laser beams. The polarised vacuum then behaves like a dielectric medium, with the generation of a fourth beam that has a distinct wavelength and direction. This will be the first tests of real photon-photon scattering in the laboratory. It might provide tests of physics beyond the standard model, including quantum gravity. The project will start in April 2024. The student will work on quantum physics that these extraordinary conditions enable.
Exploring many-body quantum physics with ultracold atoms
Ultracold atoms are a highly versatile platform for studying quantum many-body physics as both the trapping geometry and the inter-particle interactions can be controllably tuned. Our experiments are based on ultracold erbium atoms which feature large magnetic dipole moments, this result in long-range and anisotropic dipole-dipole interactions in addition to the short-range contact interactions more normally seen in cold atom systems. These interactions can fundamentally change how a system behaves, for example they can trigger a transition from a Bose-Einstein Condensate to a supersolid phase (which is both a superfluid and also features the spontaneous long-range order normally associated with a solid). Examples of projects include:
Single impurity in a dipolar Bose-Einstein condensate
A single impurity interacting with a quantum bath is a simple (to state) yet rich many-body paradigm that is relevant across a wide sweep of fields from condensed matter physics to quantum information theory to particle physics. The aim of this project is to experimentally explore this physics using the highly controllable platform of an ultracold bath of Erbium atoms in which potassium impurities can be imbedded. This opens new avenues in a range of topics from polaron physics to information flow in open quantum systems.
Out-of-equilibrium many-body quantum systems
The scientific understanding of non-equilibrium phenomena is generally less advanced than that of the related equilibrium states. Consider for example an isolated many-body quantum system; there are many questions that are still far from answered: What determines whether a quantum system will equilibrate? How does a quantum system equilibrate? Does equilibration always mean thermalisation? What is the role of temperature? Topics to be covered will include studying the effect of long-range interactions on critical phase transition dynamics and studies of periodically driven systems and turbulence.
Exploring Quantum Plasmas with X-ray Free-Electron Lasers
The advent of high-brightness 4th generation free-electron laser (FEL) light sources has revolutionised our ability to study extreme states of matter with unprecedented precision and control. The addition of new high-repetition rate, high energy laser drivers to FEL beamlines, such as the Dipole laser at the high-energy-density (HED) endstation of the European XFEL, will allow for a host of new compression experiments in well-controlled high-energy-density conditions to be investigated. In particular, the capability to tune the inter-atomic spacing between atoms in plasmas and compressed solids to the point where inner-shell electrons start overlapping, interacting and hybridizing is of great interest as it constitutes a new quantum frontier in dense plasmas. This novel regime is one where quantum effects and correlation may be sustained up to very high temperatures, and can now be accessed for the first time in the laboratory.
DPhil projects exploring this quantum plasma regime are available, with a focus on theoretical, computational, or experimental research.
Our experimental efforts are undertaken at large-scale FEL facilities, such as LCLS in California and the European XFEL in Hamburg, where we deploy a range of spectroscopy and x-ray diffraction techniques to understand how high-energy-density systems can be generated, and how they behave in extreme conditions of temperature and pressure. For a flavour of the sort of work we do, some of our recent papers in this area are:
- Mapping the Electronic Structure of Warm Dense Nickel via Resonant Inelastic X-ray Scattering.
- Time-Resolved XUV Opacity Measurements of Warm Dense Aluminum.
- Clocking Femtosecond Collisional Dynamics via Resonant X-Ray Spectroscopy.
Our experimental work closely ties into computational modelling (density functional theory, collisional-radiative atomic kinetics), and the application of advanced statistical tools and machine learning to help interpret complex experimental measurements in large-dimensional spaces:
- Building high accuracy emulators for scientific simulations with deep neural architecture search. Also see reports on this work in Nature Physics and Science.
- Ab initio simulations and measurements of the free-free opacity in aluminum.
- Inverse problem instabilities in large-scale modelling of matter in extreme conditions.
Searching for the Universal Functional with Differentiable Density Functional Theory
Kohn–Sham density functional theory (KS-DFT) is among the most popular approaches to modelling the quantum electronic structure of molecules and extended systems, and is widely used across physics, chemistry and material science. It is an exact formulation of the full quantum manybody problem, but in practice many approximations are needed to treat the very challenging electron exchange and correlations (XC) effects. Central to the theory is the existence of a universal functional – one independent of potentials external to the manybody electron system. This functional incorporates all the XC effects and can thus model complex physical systems exactly, with the added benefit of a low computational cost typical reserved for less accurate mean field approximations. Alas, this universal functional, also know as the exact XC functional, remains unknown.
Recent developments in our research group have shown how the XC functional can be efficiently modelled by a deep neural network embedded within a fully-differentiable KS DFT framework. Remarkably, this approach allows us to use experimental observables directly from nature to infer the properties of the XC functional in a way that transcends specific materials, elements, and properties.
This project will make use of these groundbreaking early developments to further shape our understanding of the XC functional and its impact on the prediction of complex quantum properties for advanced materials discovery. Work will include adding further constraints to train and validate the deep-learned XC neural network, extending code capabilities to larger systems, modelling dynamical properties, and designing materials based on required physical or chemical properties.
Candidates should be familiar with and interested in writing and modifying software, coding in python (including PyTorch), and using automatic differentiation. A solid background in atomic and/or condensed matter physics will be beneficial.
Relevant references
2. DQC: a Python program package for Differentiable Quantum Chemistry
Equation of state and structure of matter at extreme densities
Professor Sam Vinko and Professor Justin Wark
Density functional theory combined with classical molecular dynamics (DFT-MD) is a key workhorse for equation of state (EoS) calculations of matter in extreme conditions. However, as the density is increased, quantum effects, and electron correlations are expected to become increasingly important in predicting how the system will evolve, and what its structure will be. Within the DFT-MD paradigm these effects are determined by the exchange-correlation functional (XC), and the popular libxc library lists over 400 functionals to choose from. The importance of this choice is to some degree determined by the system and conditions of interest, but there remains no systematic way to predict which XC will be more accurate, especially for high density systems that are central to the modelling of exoplanetary systems.
In this project the student will start addressing these challenges making use of cutting-edge DFT simulations, and combining them our newly developed differentiable DFT suite DQC (Differentiable Quantum Chemistry). The project will explore how the choice of XC functional affects the predicted EoS of matter in extreme conditions, with a particular focus on systems of interest to exoplanetary science (H2O, CO2, CH4, mixtures, iron complexes etc.). We will investigate how our tools can be used to improve XC functionals to maximize the predictive capability of DFT simulations in predicting the EoS, structure and transport properties of matter in extreme conditions, opening new avenues for exploratory HED science.
Subject to approval this studentship will be supported by the Oxford Centre for High Energy Density Science (OxCHEDS) in partnership with AWE, and will be fully funded for UK students with a significantly supplemented DPhil stipend. Further details can be provided upon request.
Relevant References:
[1] Kasim & Vinko, Learning the exchange-correlation functional from nature with fully differentiable density functional theory. PRL 127, 126403 (2021).
[2] Kasim, Lehtola, Vinko, DQC: a Python program package for Differentiable Quantum Chemistry. Journal of Chemical Physics 156, 084801 (2022).
[3] Azadi, Drummond, Vinko, Correlation energy of the paramagnetic electron gas at the thermodynamic limit. https://arxiv.org/abs/2209.10227 (2022).
Two projects: Multi-messenger measurements of the equation of state of impact-fusion materials and Microscopic properties of fusion materials using Molecular Dynamics
Professor Gianluca Gregori and Prof. Sam Vinko
1.Multi-messenger measurements of the equation of state of impact-fusion materials
We want to experimentally study the properties of impact fusion materials using complementary and independent diagnostics. We are particularly interested in warm dense matter (WDM), which is challenging to model as it both exhibits long-range correlations as well as quantum effects. Here we propose to create WDM states using high-power lasers and then probe them with exquisite accuracy with an X-ray Free Electron Laser. By implementing simultaneously X-ray diffraction, X-ray scattering (both non-resonant and resonant), as well as X-ray photo-correlation spectroscopy and velocity interferometry, and exploiting the strengths and limitations of each diagnostics, the student on this project will be able to develop a novel experimental platform, where reduction of model selection biases is obtained by cross-validation across multiple diagnostics. The experimental data will be used to inform numerical simulation models and validate the theoretical understanding of WDM. This will be important not only for the success of impact fusion but also for the modelling of planetary interiors and white dwarfs, which all share close similarities with laboratory WDM systems.
The student will join a dynamic research environment working under the UKRI Prosperity Partnership programme in fusion energy, bringing together several UK Universities (Oxford, Imperial College and York) as well private companies (First Light Fusion and Machine Discovery).
Background Reading:
- https://journals.aps.org/prresearch/abstract/10.1103/PhysRevResearch.6.023144
- https://pubs.aip.org/aip/pop/article/29/7/072703/2844279/A-case-study-of-using-x-ray-Thomson-scattering-to
2.Microscopic properties of fusion materials using Molecular Dynamics
This studentship will explore static and dynamic properties of impact fusion materials under extreme conditions. We expect that such materials will achieve both large densities and temperatures where many body correlations, quantum de-localization and relativistic effects are all important. To address these challenges we will employ a novel simulation approach using a recently developed massively-parallel Molecular Dynamic code. The student is expected to develop equation of state tables as well as calculate relevant transport coefficient for that can be used in radiation hydrodynamics simulations of impact fusion experiments. We expect the student to benchmark and validate the calculations against experimental data and/or detailed ab-inito simulations (using DFT-MD where possible). The student will join a dynamic research environment working under the UKRI Prosperity Partnership programme in fusion energy, bringing together several UK Universities (Oxford, Imperial College and York) as well private companies (First Light Fusion and Machine Discovery).
Background Reading:
Modelling macroscopic free-energy sources in high-energy-density plasmas via anomalous microphysical forces
Dr Archie Bott and Prof Gianluca Gregori
There is a pressing need to establish new theories that relate macroscopic free-energy sources (e.g. temperature gradients) to transport properties (e.g. heat conductivity) in the hot, dense plasmas commonly present in high-energy-density (HED) physics experiments [1,2]. Measurements of laser-produced plasmas have shown that classical models of transport properties, which only consider the effect of Coulomb collisions between a plasma’s constituent particles, often fail when applied to HED plasmas [3]. This finding, which it is theorised is due to neglecting collective plasma interactions [4], is particularly unfortunate for inertial-confinement-fusion (ICF) research because accurate modelling of heat transport is crucial for realising high-gain target designs. Other research groups have recently attempted to address this problem using particle-in-cell (PIC) simulations, e.g. [5-7]; however, these previous efforts only focus on astrophysically relevant plasma conditions and consider specific free-energy sources that cannot be generalised. In this project, a student would learn to use a newly proposed numerical approach that overcomes the latter limitation by modelling free-energy sources in PIC simulations via ‘anomalous’ velocity-dependent forces applied to particles, and then apply it to HED and ICF-relevant plasmas.
Key reading:
1. R.S. Craxton et al, Phys. Plasmas 22, 110501 (2015)
2. M.A. Barrios et al, Phys. Rev. Lett. 121, 095002 (2018)
3. J. Meinecke et al, Sci. Adv. 8, eabj6799 (2022)
4. A.F.A. Bott et al, J. Plasma Phys. 90, 975900207 (2024)
5. S. Komarov et al, J. Plasma Phys. 84, 905840305 (2018)
6. J.F. Drake et al, Astrophys. J. 923, 245 (2021) 7. E. Yerger et al, arXiv:2405.06481 (2024)
Explaining the suprathermal ion populations produced in ignited inertial-confinement-fusion plasmas
Dr Archie Bott and Prof Gianluca Gregori
Recent indirect-drive inertial-confinement-fusion (ICF) experiments at the National Ignition Facility (NIF) have, for the first time, demonstrated ignition in a controlled fusion experiment [1,2]. In such experiments, the conditions achieved at the centre of the deuterium-tritium capsule (‘the hot spot’) during its maximum compression are sufficiently hot and dense for heating by fusion-produced alpha particles to overcome other cooling mechanisms, leading to the possibility of high energy gain [3]. One surprising observation from these experiments is a discrepancy between measured neutron spectra and what would be expected if the plasma’s underlying ion distribution function obeyed Maxwellian-Boltzmann statistics. Instead, the data suggests that a robust population of suprathermal ions is produced [4]. In this project, a student would investigate various possible explanations for this phenomenon, and in particular, acceleration due to alpha-particle-driven kinetic plasma instabilities and dynamics. Understanding the cause of this discrepancy could be vital for realising robust ICF implosions with target gain well beyond unity, and their accurate modelling.
Key reading:
1. H. Abu-Shawareb et al, Phys. Rev. Lett. 129, 075001 (2022)
2. H. Abu-Shawareb et al, Phys. Rev. Lett. 132, 065102 (2024)
3. A. Zylstra et al, Nature 601, 542 (2022)
4. E.P. Hartouni et al, Nature Phys. 19, 1 (2022
Effective Transport Coefficients in Extreme Dynamic Materials
Professor Gianluca Gregori and Prof. Andrei Starinets
Characterizing and quantifying mass, momentum, and energy transport in materials under extreme conditions is vital in many areas of research, ranging from inertial confinement fusion to the behaviour of matter in the interiors of giant planets and stars. With temperatures of a few electron volts (eV) and densities comparable to solids, warm dense matter (WDM) forms a key constituent of planetary interiors [1] as well as cooler stellar objects such as brown dwarfs and the crust of neutron stars [2]. WDM is also produced during laser processing of solids and is an important transient state in all approaches to inertial confinement fusion [3] (ICF). Transport properties are difficult to model in WDM [4]. Our goal here is to develop a theoreticall and numerical framework that can be used to calculate effective transport in WDM and then use the experimental data to construct a suitable representation via symbolic regression or a trained neural network [5].
Our proposed work utilizes recent advances in string theory and in machine learning.
We seek a deeper understanding of transport properties of strongly coupled matter in general by applying a new modelling approach known as holography (or gauge-gravity duality) [6]. This method has been developed to compute correlation functions and transport coefficients of strongly interacting quantum systems at finite temperature and density for a class of systems where the dual description is known to exist. Holography refers to the mapping between a d-dimensional quantum field theory and a (d+1)-dimensional dual. What is fascinating is that the dual of a strongly coupled field theory is weakly coupled, and so it can be treated via perturbation theory. By transforming back, properties of the strongly coupled system can then be retrieved. In plasmas, these techniques have only be applied in determining the ratio of of the shear viscosity to entropy density in the limit of infinitely strong coupling, and validation under finite coupling conditions is needed. Here we want to propose a novel machine learning approach to address the complex micro-physics of material strength properties and to identify their emergent behaviour via closed mathematical expressions. This is done by using a Graph Neural Network[7] (GNN) to represent the discrete description of the underlying continuum system and then applying deep learning techniques to obtain a representation of the material properties as a function of the state variables (density, temperature, etc.) The latent representation learned by the GNN is then extracted with a symbolic regression analysis [8]. Our long-term goal is the development of augmented methods to ultimately improve the design and verification integrated modelling of WDM systems, in the sense that fluid simulations using these effective transport coefficients may now be able to capture the relevant physical processes at all scales.
One of the student’s task will consist in participating in experiments at Free Electron Laser facilities and develop an experimental diagnostics able to extract transport coefficients such as mass diffusion, thermal conduction or viscosity. Once a sufficiently large database has been obtained, the student will then train the GNN and apply symbolic regression techniques in order to extract an effective representation of those transport coefficients.
This project is fully funded and we are looking for interested students in pursuing this line of research.
Background Reading:
1. Guillot, T., 1999: Science, 286 (5437), 72–77.
2. Ichimaru, S., 1982: Rev. Mod. Phys., 54 (4), 1017–1059.
3. Hurricane, O. A. et al., 2016: Nat. Phys., 12 (8), 800–806.
4. Grabowski, P. et al., 2020: High Energy Density Physics, 37, 100 905.
5. Miniati F. and Gregori, G. 2022: Sci. Rep., 12, 11709.
6. Son D. T., and Starinets, A. O. 2007: Annu. Rev. Nucl. Part. S , 95.
7. Battaglia P. W. et al., 2018: Arxiv:1806.01261v3 (2018).
8. Udrescu, S.-M. and Tegmark, M., 2020: Sci. Adv. 6, eaay2631.
Theory of many-body quantum optics, quantum computing and quantum simulation
Over the past two decades there has been transformative growth in available experimental platforms with control over single atoms, molecules, spins, or photons. There are now quantum computing systems with neutral atoms in tweezer arrays and superconducting circuits that have single-particle control of hundreds of qubits, and related high-precision systems with trapped ions and solid state spins. These systems present both opportunities and challenges for theoretical physics. The opportunities come because we can explore physical phenomena that were previously inaccessible, especially asking major questions about out of equilibrium dynamics, and strongly interacting many-particle systems. The challenges come in understanding how to control noise and decoherence, how to enter and verify regimes of quantum advantage over classical computation, and how to apply these systems to the most interesting computational problems in science and beyond.
Our theory team uses analytical and numerical techniques to explore the opportunities and address challenges set by these experiments. Our primary aims are (1) to explore novel physical phenomena that can be studied in these experiments, motivated by new experimental techniques and opportunities, and (2) to set a roadmap for experiments, providing new potential architectures and applications for quantum computing and quantum simulation of many-body systems. We also work directly with experimental teams locally, nationally, and internationally to realise parts of this roadmap, and directly model what is seen in the laboratory.
Our open specific projects include:
Exploration of dynamics in systems with long-range interactions:
Several experimental platforms allow for the generation of long-range interactions (atoms in optical cavities, arrays of trapped ions, and moving atoms in tweezer arrays). Recently, we have begun to explore how this gives rise to fast scrambling of quantum information, a phenomenon that was originally discussed in the context of quantum information in black holes. This opens interesting connections to many-body physics in black holes, while providing a route in experiments to rapid generation of entanglement, and potentially resource states for quantum computing. We recently identified a dynamical phase transition that occurs as a function of the range of the coupling distance, between regimes of slow and fast scrambling. We plan to explore the many-body physics of this further, including looking at ground-state phase transitions as a function of the coupling strength, which could be explored in a series of ongoing experiments.
· Nicolò Defenu, Tobias Donner, Tommaso Macrì, Guido Pagano, Stefano Ruffo, and Andrea Trombettoni
Rev. Mod. Phys. 95, 035002 (2023)
· Tomohiro Hashizume, Gregory S. Bentsen, Sebastian Weber, Andrew J. Daley, Deterministic Fast Scrambling with Neutral Atom Arrays, Phys. Rev. Lett. 126, 200603 (2021).
· Gregory Bentsen, Tomohiro Hashizume, Anton S. Buyskikh, Emily J. Davis, Andrew J. Daley, Steven S. Gubser, and Monika Schleier-Smith, Treelike interactions and fast scrambling with cold atoms, Phys. Rev. Lett. 123, 130601 (2019).
· Sridevi Kuriyattil, Tomohiro Hashizume, Gregory Bentsen, and Andrew J. Daley, Onset of scrambling as a dynamical transition in tunable-range quantum circuits, PRX Quantum 4, 030325 (2023)
Theory of quantum computing and simulation with neutral atoms
Neutral atom arrays have rapidly emerged as a leading candidate for implementation of general purpose quantum computing, and continue to be a leading candidate in analogue quantum simulation. We will explore the new opportunities provided by (1) the ability to move atoms in the array during the dynamics, especially creating hypercube architectures for quantum computing, (2) new ways to verify quantum advantage in analogue quantum simulation. We will also explore more deeply how decoherence that is present in experiments, and which we can model on a microscopic level, changes our ability to compute the dynamics using classical numerical techniques, shifting the criteria for achieving a quantum advantage.
· D. Bluvstein et al., Nature 604, 451 (2022)
· Andrew J. Daley, Immanuel Bloch, Christian Kokail, Stuart Flannigan, Natalie Pearson, Matthias Troyer, and Peter Zoller, Practical quantum advantage in quantum simulation, Nature 607, 667 (2022).
· S. Flannigan, N. Pearson, G. H. Low, A. Buyskikh, I. Bloch, P. Zoller, M. Troyer, A. J. Daley, Propagation of errors and quantitative quantum simulation with quantum advantage, Quantum Science and Technology, 7, 045025 (2022).
· Gerard Pelegrí, Andrew J. Daley, Jonathan D. Pritchard, High-fidelity multiqubit Rydberg gates via two-photon adiabatic rapid passage, Quantum Science and Technology, 7, 045020 (2022).
Many-body quantum optics and open quantum systems
Control over individual quantum particles was originally pioneered from the point of view of quantum optics (resulting in the Nobel prize for Serge Haroche and Dave Wineland in 2012). There has been a long tradition of exploring continuous measurement techniques, and understanding how they can be used to control a many-particle quantum system. We recently developed new methods that allow us to compute the state of many-particle quantum systems undergoing non-markovian dissipative processes, as arises, e.g., with atomic ensembles in an optical cavity. We will explore generalisations of these ideas, both to understand (1) how measurement and feedback in multi-mode optical cavities can be used to engineer chosen many-body states with applications to generating resource states for quantum metrology and quantum computing, and (2) how quantum optics techniques can be generalised to provide descriptions of modern quantum transport systems.
· Valentin Link, Kai Müller, Rosaria G. Lena, Kimmo Luoma, François Damanet, Walter T. Strunz, and Andrew J. Daley, Non-Markovian Quantum Dynamics in Strongly Coupled Multimode Cavities Conditioned on Continuous Measurement, PRX Quantum, PRX Quantum 3, 020348 (2022).
· Stuart Flanningan, François Damanet, and Andrew J. Daley, Many-body quantum state diffusion for non-Markovian dynamics in strongly interacting systems, Phys. Rev. Lett. 128, 063601 (2022).
· A. J. Daley, Quantum trajectories and open many-body quantum systems, Adv. Phys. 63, 77 (2014). (arXiv:1405.6694)
· Inés de Vega and Daniel Alonso, Rev. Mod. Phys. 89, 15001 (2017)
Quantum network switch based on single ion control
Dr. Gabriel Araneda and Prof. David Lucas
Trapped atomic ions present an ideal platform for quantum computing due to their long coherence times, precise control, and inherent qubit connectivity. These systems have already demonstrated the functionality of small, fully controllable universal quantum computers. However, scaling remains a significant challenge. As the number of qubits increases, control becomes more difficult due to factors like single-qubit addressing and spectral crowding. Solving this challenge is critical, as most practical applications require a larger number of qubits to achieve "quantum advantage" — where quantum computers outperform classical ones in specific tasks. This scaling challenge is not unique to ion-based systems but affects all quantum computing platforms.
One potential solution is to interconnect discrete, fully controllable quantum processor modules, rather than concentrating all qubits in a single processor. Two approaches have been proposed to enable this connection. The first, known as "QCCD", involves physically moving ions between different processing zones during computation. The second approach uses photonic entanglement, where single photons emitted by ions create quantum connections between different processors. Photonic entanglement allows for flexible, long-distance connections with minimal decoherence, and photons can be routed to connect any two processors in a network, not just adjacent ones, allowing for highly adaptable quantum networks.
Our team at the University of Oxford has developed one of the world’s most advanced ion trap network. This pioneering system, comprising two separate ion trap processors, has successfully demonstrated proof-of-principle protocols for distributed quantum computing [1], blind quantum computing [2], quantum communications [3], and remote quantum sensing [4]. While a two-node network is sufficient for these initial experiments, it is far from enough to prove the scalability required for practical quantum computing. In this project, we aim to advance the network’s capabilities by developing the techniques and technologies that will allow for distributing entanglement across multiple nodes. Demonstrating distribution of entanglement between a greater number of remote systems is crucial to showcasing the true potential of scalable quantum networks, laying the groundwork for practical quantum technologies.
In this project, we aim to develop two key techniques and peripherals that will enable the distribution of entanglement between multiple entangling nodes: a photon collection unit and a entanglement switch. The project is part of a collaboration with the National Quantum Computing Centre (NQCC).
References
[1] D. J. Main et al., arXiv:2407.00835 (2024)
[2] P. Drmota et al., PRL 132, 150604 (2023)
[3] D. P. Nadlinger et al., Nature 607, 682 (2022)
[4] B. C. Nichol et al., Nature 609, 689 (2022)
This position is a collaborative studentship between the University of Oxford and the National Quantum Computing Centre (https://www.nqcc.ac.uk). The position will be registered and hosted at the university, within the group of Prof. David Lucas. The student will also have a co-supervisor at the NQCC who is an expert in the field. Over the course of the studentship students will be offered a minimum of three months to work at the NQCC with relevant research teams. This position is part of a wider cohort of 6 collaborative studentships, in which the projects have been co-developed by the NQCC and different academic institutes across the UK. The scheme will include cohort-based training and activities, enabling students to gain wider skills and develop valuable personal and professional networks.
One Wire Quantum Computing
Dr. Mario Gely and Prof. David Lucas
Quantum computing (QC) promises an exponential increase in computing power for a range of applications spanning from energy efficiency to drug discovery. Of all approaches to QC, trapped ions boast the highest fidelity operations, but the path for scaling to a larger number of qubits remains clouded.
The most widely explored approach is to use microfabricated chips enabling the transport of ions to interaction zones, where logical operations are driven on pairs of qubits by lasers. Hundreds of voltages must be controlled on-chip to transport ions without disturbing them, and high-power laser beams must be focused on the ions with carefully stabilised amplitude and phase. This complexity means 10-ion systems are out of reach of small university-based experiments and well-resourced companies remain limited to systems with 10’s of ions. In this project, we take on the challenge of simplifying these systems to such an extent that today’s large-scale experiments become simple university lab setups, with a clear path to scale further.
To this end, we are exploring a new technique we call One-Wire Quantum Computing. In this technique, a single wire, or rather an on-chip electrode, will generate the microwave field required to control a chain of ions. Indeed, microwaves can provide global entangling interactions (natively suited to their long wavelength), which can be combined with the best single-qubit addressing of any QC platform (demonstrated at Oxford), to implement arbitrary quantum algorithms.
This project will extend the state-of the art microwave-control methods developed at the Oxford ion trapping group. In this field the group holds several records and world firsts: the first ion trap to incorporate integrated microwave circuit elements [1]; the first ever high-fidelity (>99%) microwave driven two qubit gates [2]; the highest fidelity single-qubit gates of any quantum computing platform [3]; and the highest fidelity addressed single-qubit gates of any quantum computing platform [4]. These technical abilities are at the core of the proposed project. The project is part of a collaboration with the UK National Quantum Computing Centre (NQCC) and the US quantum computing program.
[1] D T C Allcock et al. New J. Phys. 12 053026 (2010)
[2] T P Harty et al. Phys. Rev. Lett. 117, 140501 (2016)
[3] M C Smith et al. Manuscript in preparation
[4] Leu et al. Phys. Rev. Lett. 131, 120601 (2023)
This position is a collaborative studentship between the University of Oxford and the National Quantum Computing Centre (https://www.nqcc.ac.uk). The position will be registered and hosted at the university, within the group of Prof. David Lucas. The student will also have a co-supervisor at the NQCC who is an expert in the field. Over the course of the studentship students will be offered a minimum of three months to work at the NQCC with relevant research teams. This position is part of a wider cohort of 6 collaborative studentships, in which the projects have been co-developed by the NQCC and different academic institutes across the UK. The scheme will include cohort-based training and activities, enabling students to gain wider skills and develop valuable personal and professional networks.
Ion trap-integrated optical cavities for fast networked quantum computation
Dr Joe Goodwin (PI/ERC Fellow)
PDRA support: 3x dedicated project PDRAs
Distributed networks of small quantum processor nodes offer a compelling route to scalability, with ions in remote nodes entangled via single photons emitted from each ion and transmitted over optical fibre. However, the performance of ion-photon networks is typically limited by the low efficiency of lens-based photon collection. This introduces a bottleneck to network speed which makes the approach unsuitable for scaling. An alternative is to use optical cavities, which enable near-unit collection efficiency into low NA, making them compatible with most trap geometries while offering much higher performance than refractive optics. It is widely recognised that efficient quantum networks will require cavity-based interfaces, and there is a strong imperative to realise the full potential of this approach via a reliable and robust design.
Networked architectures also reduce the number of qubits required for useful computation, by virtue of their high connectivity. As quantum processors grow in size, the ability to perform gates directly between any pair of qubits will represent a key advantage over monolithic platforms, most of which permit only nearest-neighbour gates. Crucially, the long-range connectivity provided by a photonic network opens a door to new techniques for quantum error correction, offering the prospect of greatly reduced qubit overheads and improved resilience to noise, which could accelerate progress towards the formidable goal of fault tolerant quantum computation.
The MICRON-QC project will build the first reconfigurable network of trapped ion processors linked by single photons, ultimately consisting of 5 nodes. We will demonstrate all elements needed for efficient large-scale networked computation including: a flying qubit suitable for high-fidelity long-range transmission; a reconfigurable network enabling any-to-any node connectivity; and the functionality in each node required to assemble arbitrary entangled network graph states. We will construct cavity-based network interfaces at each node yielding near-deterministic ion-photon entanglement and allowing remote ion-ion entanglement creation at 100kHz rates, close to that of local gates. While the 5-node network constructed in this project will enable many fascinating experiments, the principal objective will be to prove that a network of hundreds or more nodes is within reach.
In recent years our team has pioneered a number of ground-breaking approaches to the design and construction of ion-trap network nodes, which will integrate passively aligned microcavities and other functional modules within the structure of the ion trap chip itself. Combined with novel approaches to packaging and optical delivery, this will yield nodes of simple, robust construction, enabling near-autonomous operation. The student would join at an exciting stage, with the supporting infrastructure and experimental systems in place. They would begin by making significant contributions to the project in characterising the performance of one or more ion trap network node designs and using one of these systems to produce high-rate ion-photon entanglement. In their second and third years, they would help build additional nodes and use these to demonstrate remote ion-ion entanglement at rates comparable to those of local gates, a milestone result for networked quantum computing.